Converting DNA Sequences to Amino Acids: A Comprehensive Guide
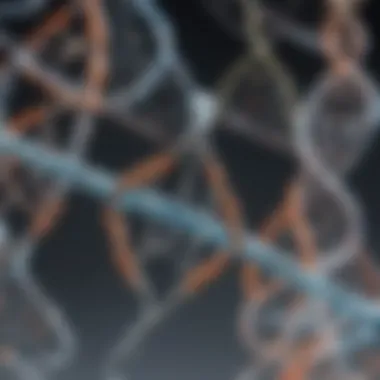
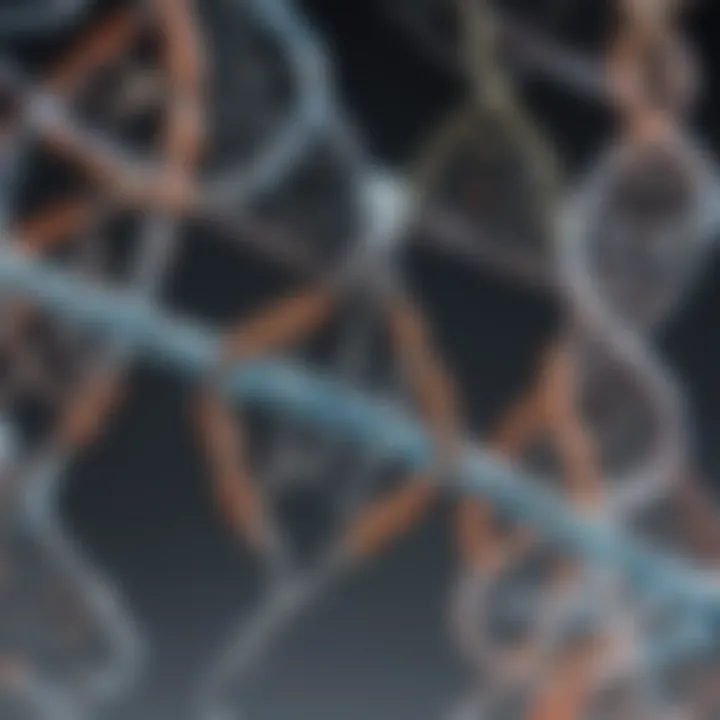
Intro
The conversion of DNA sequences to amino acids is a crucial process that is fundamental to the workings of all living organisms. At the heart of this topic lies the genetic code, which serves as the bridge between deoxyribonucleic acid and protein synthesis. Every aspect of cellular function hinges on proteins, which are responsible for a multitude of activities within cells. This process, though intricate, is essential for all advanced biological functions.
Understanding how DNA translates into amino acids not only sheds light on the principles of molecular biology, but it also unveils the implications of genetic variations and mutations. In this guide, we delve into the various phases of this conversion process while highlighting the importance of each step.
As we move through this article, we will explore several key concepts, including the roles of RNA, the significance of codons, and the mechanisms that drive translation. By the end of this guide, readers will have a clear understanding of how genetic information translates into functional proteins. This knowledge is vital, offering insights into both evolutionary biology and modern biotechnological applications.
Prolusion to DNA and Amino Acids
Understanding the transformation of DNA sequences into amino acids is vital in the field of molecular biology. This process underlies the functionality of proteins, which are essential macromolecules in all living organisms. The conversion of genetic information into functional proteins forms the basis of numerous biological processes including metabolism, cellular signaling, and immune responses.
The significance of this discussion lies not only in grasping how information is encoded in DNA but also how it orchestrates the synthesis of proteins that carry out various tasks in the cells. Furthermore, with advancements in biotechnology and genetic engineering, a deep comprehension of DNA and amino acids can influence medical research and the development of therapeutics, making it increasingly relevant today.
The Role of DNA in Biological Systems
DNA serves as the blueprint for life. It contains the instructions necessary for the development and functioning of all living organisms. The structure of DNA, comprising two strands that coil to form a double helix, is composed of nucleotides, each consisting of a sugar group, a phosphate group, and a nitrogenous base. The sequence of these nitrogenous bases determines the genetic information carried by the DNA.
In biological systems, DNA is responsible for processes such as replication, where genetic material is copied for cell division. Additionally, DNA plays a critical role in transcription, the process of synthesizing messenger RNA (mRNA), which carries genetic information from the DNA to ribosomes for protein synthesis. The integrity of this DNA is paramount as any mutations or alterations can lead to detrimental effects on organismal health and function.
Understanding Amino Acids
Amino acids are the building blocks of proteins. Each protein is made up of a specific sequence of amino acids, dictated by the mRNA. There are 20 standard amino acids, each with distinct characteristics that influence the structure and function of the resulting proteins.
Amino acids are categorized based on their side chains into nonpolar, polar, and charged groups. This classification affects how amino acids interact with each other and fold into three-dimensional shapes that define protein functionality. Essential amino acids must be obtained through diet, while non-essential amino acids can be synthesized by the body.
Understanding the role of amino acids is crucial because changes in their sequence or structure can lead to significant alterations in protein function. For instance, a single amino acid substitution can result in a condition such as sickle cell disease, highlighting the importance of precision in genetic coding and protein synthesis.
The Genetic Code: An Overview
Understanding the genetic code is essential for grasping how DNA sequences translate into functional proteins. The genetic code consists of a set of rules that dictate how nucleotide sequences in DNA correspond to amino acids, which are the building blocks of proteins. This section will discuss the nature and components of the genetic code, outlining their significance in the process of protein synthesis.
Nature of the Genetic Code
The genetic code is inherently universal, which means it is nearly the same across all living organisms. This universality suggests a common evolutionary origin. The genetic code operates on a triplet system, where sequences of three nucleotides, called codons, specify a particular amino acid. This arrangement allows for 64 possible codons, even though only 20 amino acids are commonly used in the synthesis of proteins. This redundancy ensures that minor mutations usually do not affect protein function; a phenomenon known as degeneracy.
Components of the Genetic Code
The genetic code can be broken down into several key components, which facilitate the accurate translation of genes into proteins.
Codons
Codons are crucial to the genetic code as they directly correlate with amino acids. Each codon consists of three nitrogenous bases. The unique feature of codons is their ability to code for multiple amino acids, which allows for flexibility in protein synthesis. This aspect makes codons a beneficial choice for maintaining genetic stability amidst potential mutations. In practice, the redundancy of codons can mitigate the impact of a point mutation, contributing to evolutionary resilience.
Start and Stop Codons
Start and Stop codons play critical roles in signaling the beginning and end of protein synthesis. The key characteristic of a start codon is that it usually signals the commencement of translation, with the most common one being AUG, which codes for methionine. The unique feature of stop codons, such as UAA, UAG, and UGA, is that they do not code for any amino acid but instead trigger the termination of the protein chain. This distinction is vital for ensuring that the protein elongates properly and does not run on indefinitely.
Together, these components of the genetic code facilitate the accurate translation of genetic information into functional proteins, a process that is foundational to all forms of life. Understanding these mechanisms not only enriches our comprehension of molecular biology but also opens avenues for advancements in genetic engineering and biotechnological applications.
From DNA to mRNA: Transcription Process
The transcription process is a critical step in the flow of genetic information from DNA to proteins. In this process, the DNA sequence is used to synthesize messenger RNA (mRNA), which carries the genetic information necessary for protein synthesis. Understanding this topic is essential for grasping subsequent processes like translation. It marks the transition between the static genetic code in DNA and the dynamic functions of proteins that shape biological systems. This section will delve into the mechanisms of transcription along with the modifications mRNA undergoes after synthesis.
Mechanisms of Transcription
Transcription begins with the unwinding of the DNA double helix, exposing the template strand. RNA polymerase, the key enzyme, binds to a specific region of the DNA called the promoter. This binding is crucial as it determines where transcription starts.
Once the RNA polymerase is attached, it begins synthesizing a complementary RNA strand by adding ribonucleotides that match the DNA template.
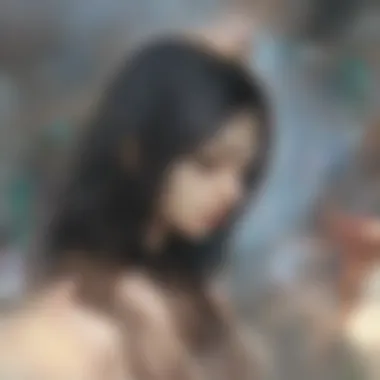
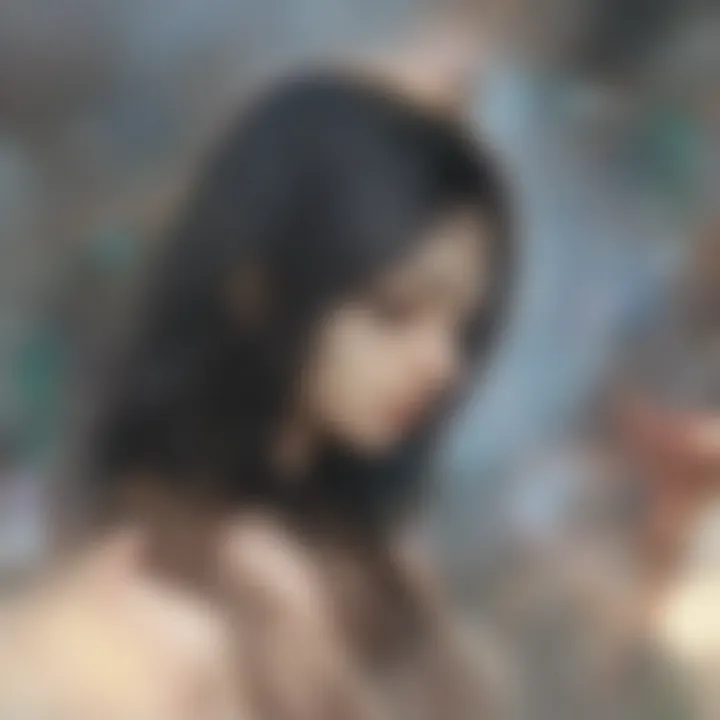
- Initiation: In this phase, the RNA polymerase binds to the promoter and separates the DNA strands. This creates a transcription bubble.
- Elongation: As RNA polymerase moves along the DNA, it incorporates ribonucleotides one by one, extending the RNA strand. The enzyme adds nucleotides complementary to the DNA template, creating a growing mRNA molecule.
- Termination: Once RNA polymerase reaches a terminator sequence, the transcription is complete. The RNA molecule detaches, and the DNA strands re-anneal.
This process must be tightly regulated to ensure that the right genes are expressed at the right time, which is essential for cellular function and development.
Post-Transcriptional Modifications
After the initial transcription has occurred, mRNA typically undergoes several modifications before it is ready for translation. These modifications are essential for mRNA stability, transport, and translational efficiency.
Capping
Capping refers to the addition of a modified guanine nucleotide at the 5' end of the mRNA molecule. This cap serves as a protective barrier against degradation by ribonucleases. The key characteristic of capping is that it is one of the first modifications that occur shortly after transcription initiation.
The capping process is a beneficial choice for the overall topic as it enhances the stability of mRNA and facilitates its recognition by the ribosome during translation. The unique feature of the 5' cap is that it is linked through an unusual 5' to 5' triphosphate bond, which is less susceptible to enzymatic cleavage. However, the process requires additional enzymatic activity and can be energy-consuming.
Polyadenylation
Polyadenylation involves the addition of a long chain of adenine nucleotides to the 3' end of the mRNA, forming what is known as the poly(A) tail. This modification is crucial for the stability of mRNA and its export from the nucleus to the cytoplasm. The poly(A) tail significantly contributes to mRNAβs longevity in the cytoplasm, allowing the mRNA to persist long enough for translation.
The primary characteristic of polyadenylation is its role in regulating mRNA stability and translational efficiency. One unique feature is that the length of the poly(A) tail can vary, which influences how long the mRNA remains stable. A disadvantage might be that excessive polyadenylation can lead to instability and degradation of mRNA if not controlled properly.
Splicing
Splicing is the process by which introns, non-coding regions of the RNA, are removed, and exons, coding sequences, are joined together. This modification is important for creating a mature mRNA molecule that accurately represents the protein-coding information of the gene.
One key characteristic of splicing is that it allows for alternative splicing, a mechanism that enables a single gene to produce multiple protein isoforms. This feature adds complexity to gene expression and functional diversity. Although splicing is a beneficial and necessary modification in eukaryotic cells, errors during splicing can lead to the production of dysfunctional proteins and may contribute to various diseases.
These post-transcriptional modifications are therefore essential for further ensuring that mRNA is stable, functional, and appropriately ready for the next critical stepβtranslation into proteins. Understanding these modifications deepens oneβs insight into how gene expression is regulated and adapted within biological systems.
Translation: The Conversion Process
The process of translation is central to understanding how genetic information in DNA is transformed into functional proteins. This critical phase occurs after transcription and involves the assembly of amino acids into polypeptide chains following the direction encoded by messenger RNA (mRNA).
Translation essentially bridges the gap between the genetic instructions stored in DNA and the actual functioning proteins that play pivotal roles in biological systems.
Ribosome Structure and Function
Ribosomes are the molecular machines responsible for protein synthesis during translation. They consist of two subunits, the large and the small, which come together to form the complete ribosome. Ribosomes are composed of ribosomal RNA (rRNA) and proteins, establishing an intricate structure that is essential for their function.
The ribosome has three key sites for tRNA attachment: the A (aminoacyl) site, the P (peptidyl) site, and the E (exit) site. This arrangement allows for the sequential addition of amino acids as dictated by the mRNA template. The ribosomal RNA plays a crucial catalytic role in forming peptide bonds between nearby amino acids, thus facilitating the formation of a protein chain.
tRNA and Its Role in Translation
Transfer RNA (tRNA) serves as the adaptor molecule in the translation process. Each tRNA molecule is specific to one amino acid and carries that amino acid to the ribosome. The structure of tRNA is unique, featuring an anticodon region that base-pairs with codons on the mRNA.
The tRNA molecule must also have an attached amino acid at its corresponding acceptor stem. This specificity ensures that the correct amino acid is inserted into the growing polypeptide chain, thereby preserving the intended amino acid sequence dictated by the mRNA.
Steps of Translation
Initiation
Initiation is the first step of translation and sets the stage for the synthesis of the protein chain. This process begins when the small ribosomal subunit binds to the mRNA molecule at a specific region known as the start codon, which is typically AUG. The initiator tRNA, carrying methionine, then attaches to this codon, establishing the first amino acid of the polypeptide chain.
A key characteristic of initiation is the formation of the initiation complex, which includes the small ribosomal subunit, the mRNA, and the initiator tRNA. This complex formation is crucial as it ensures that translation starts accurately. One advantage of this step is that it provides a checkpoint to prevent errors at the beginning of translation, which might lead to dysfunctional proteins later on.
Elongation
Elongation is the process following initiation, where the ribosome moves along the mRNA, synthesizing the polypeptide chain. In this phase, each new tRNA, corresponding to the next codon, enters the A site of the ribosome. The amino acid transferred by the tRNA is joined to the growing chain at the P site. As the ribosome translocates, the tRNA from the P site moves to the E site and exits, while the tRNA occupying the A site moves to the P site.
A significant feature of elongation is its rapid nature; it can add up to 20 amino acids per second in eukaryotes. This efficiency is critical for cellular function, as it allows for the swift production of proteins required for various biological roles. However, there is potential for errors in this phase, emphasizing the necessity for proofreading mechanisms in the ribosome.
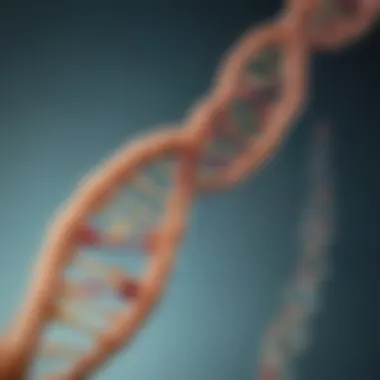
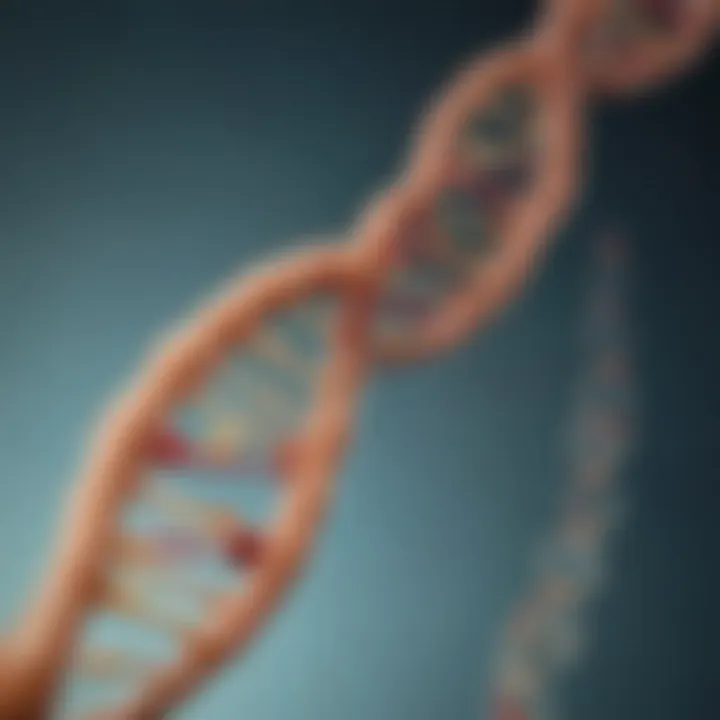
Termination
Termination occurs when a stop codon on the mRNA is reached, signaling that translation should end. Stop codons do not correspond to any amino acid but are recognized by release factors that promote the disassembly of the translation complex. The completed polypeptide chain is released, and the ribosomes detach from the mRNA.
One of the notable aspects of termination is that it ensures the proper end of protein synthesis. The release factors play a critical role, as their action prevents incomplete proteins from being produced. This step is essential for maintaining the integrity of protein function, as any errors could lead to misfolded proteins with potential harmful effects.
Codon Usage and Its Implications
Codon usage plays a critical role in the process of translating DNA sequences into functional proteins. Understanding how codon selection can influence protein synthesis is essential for anyone involved in molecular biology. This section aims to shed light on the significance of codon usage and the factors that affect it, offering insights into its practical implications in biotechnology and research.
Synonymous and Non-Synonymous Codons
Codons are triplet sequences of nucleotides that correspond to specific amino acids or signal the termination of protein synthesis. The genetic code includes synonymous codons, which are different codons that translate to the same amino acid. For example, both GCU and GCC encode the amino acid alanine. This redundancy allows for flexibility during translation and can mitigate potential errors caused by mutations.
On the other hand, non-synonymous codons lead to different amino acids. These changes can affect the structure and function of the resulting protein. Understanding the balance between synonymous and non-synonymous codon usage is essential, as it influences protein folding, stability, and overall functionality.
Impact on Protein Translation
The way codons are used in a particular organism can have significant implications for protein translation efficiency. Some codons are preferred over others, termed as 'preferred codons'. These preferred codons often correlate with high levels of tRNA molecules available in the cell. When the codons match with the available tRNA, the ribosome can translate the mRNA more smoothly, leading to a faster and more accurate protein synthesis. Conversely, less frequent codons can slow down the translation process, potentially leading to ribosome stalling and inefficiencies.
Several factors influence codon usage bias, including:
- Organism type: Different species have distinct preferences for certain codons.
- Gene function: Genes with higher expression levels often use preferred codons.
- GC content: The overall guanine-cytosine content of an organism's DNA can affect codon preference.
Understanding these factors can provide insights into gene expression and regulation. Additionally, implications are observed in biotechnology applications, where optimizing codon usage can enhance protein yield.
Effective use of codon bias is a powerful tool in synthetic biology, where engineers design genes for improved expression in host organisms.
Mutations and Their Effects
Mutations play a crucial role in the field of molecular biology. They can change the sequence of DNA, which can directly affect the resulting proteins produced. Understanding mutations is important for various reasons. For one, they can elucidate how genetic diversity arises within populations. Secondly, studying mutations helps in grasping the underlying causes of many diseases.
Types of Mutations
Point Mutations
Point mutations refer to changes in a single nucleotide base pair in DNA. They are often the simplest form of mutation. This type of mutation can be caused by various factors such as DNA replication errors or environmental influences. A key characteristic of point mutations is their ability to lead to different outcomes, ranging from benign to severe effects on an organismβs phenotype. They are a popular topic in genetic studies because they offer a clear model to study specific genetic variations.
One unique feature of point mutations is the potential to result in synonymous or nonsynonymous changes. Synonymous mutations do not alter the amino acid sequence of the resultant protein, while nonsynonymous mutations do. This indicates how subtle changes can drastically influence protein function and trait manifestation in organisms. However, it is crucial to note that not all point mutations have significant consequences. Some may even have no observable effect at all.
Insertions and Deletions
Insertions and deletions (often grouped together as indels) involve the addition or loss of one or more nucleotide pairs in a DNA sequence. They contribute to the overall genetic diversity as well. The characteristic of indels is that they can cause frameshifts, which drastically alters the downstream protein translation, potentially leading to dysfunctional proteins. This makes them a significant point of study in understanding genetic stability and mutation rates.
What differentiates insertions and deletions is their impact on gene expression. Insertions add extra bases, which can change the reading frame of the gene. In contrast, deletions remove bases, which may also lead to a reading frame shift. Both situations can severely impact an organism's biology, and they are often associated with genetic disorders. Their unique features make them a valuable tool for researchers when studying the consequences of genetic alterations.
Consequences of Mutations on Protein Function
Mutations can have varying consequences on the function of proteins produced. Some mutations can lead to a complete loss of function, while others might change the functionality of a protein, potentially conferring new traits or benefits. For instance, a mutation could lead to a protein that is more active under certain conditions, which could be advantageous in a changing environment.
However, not all mutations are beneficial. Many mutations can lead to diseases. Mutations that produce non-functional proteins are often linked to inherited disorders. Understanding the consequences of these mutations can improve our insights into disease mechanisms and inform approaches for therapeutic interventions.
"Studying mutations helps us uncover the complexities of protein interactions and their effects on health and disease."
In summary, mutations represent a double-edged sword in the realm of genetics. They are essential for evolution and adaptation, but they also pose risks that can lead to negative health outcomes. Understanding their types and consequences aids researchers in their quest to comprehend the intricacies of genetic information and its expression.
Experimental Techniques for Sequence Analysis
Understanding the conversion of DNA sequences to amino acids relies heavily on precise and accurate analysis of these sequences. Experimental techniques for sequence analysis play an essential role in this process. These techniques provide vital tools for elucidating the genetic information contained within DNA, which directly correlates with the proteins that are produced. Without these methods, the study of molecular biology would be limited in scope and accuracy.
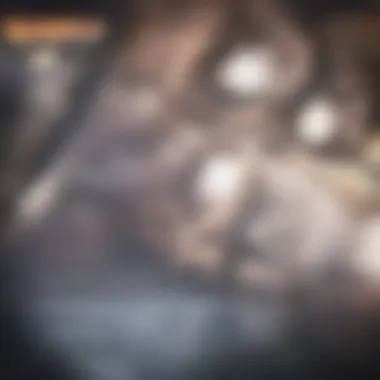
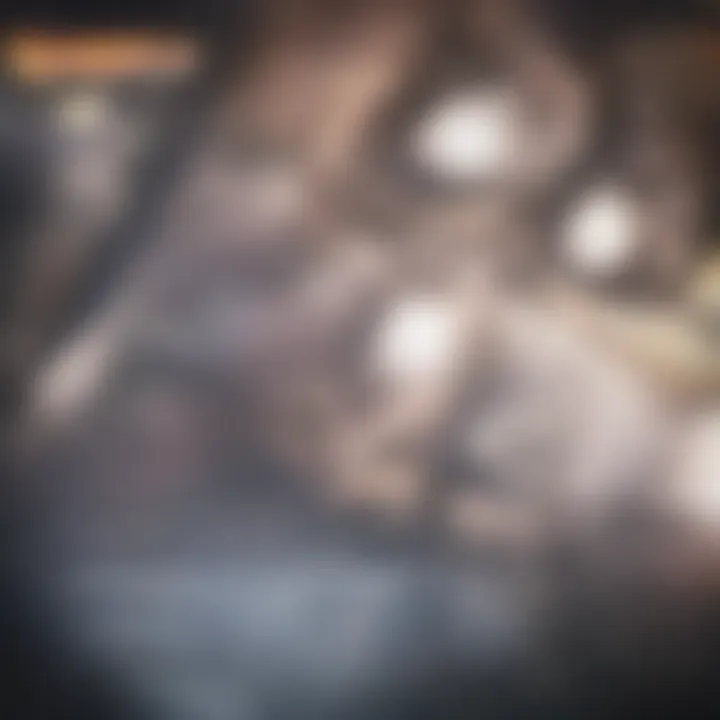
Among these techniques, DNA sequencing technologies stand out. They allow researchers to determine the exact sequence of nucleotides in a DNA molecule. Two of the most common sequencing methods are Sanger Sequencing and Next-Generation Sequencing. Each has unique traits and applications that contribute significantly to molecular biology, especially in deciphering the genetic code.
DNA Sequencing Technologies
Sanger Sequencing
Sanger Sequencing, developed in the late 1970s by Frederick Sanger, is one of the most widely used DNA sequencing methods. Its best-known attribute is the use of dideoxynucleotides, which terminate DNA synthesis at specific sites during the sequencing process. This results in a series of DNA fragments that can be analyzed to reveal the sequence of the original DNA template.
One of the main reasons Sanger Sequencing is favored is its high accuracy. This method produces reliable data which is crucial for confirming sequences in smaller, targeted studies, such as those involving known genetic mutations. It is especially useful for sequencing specific genes or regions in the genome. However, its major limitation is throughput; Sanger Sequencing is time-consuming and not practical for sequencing large genomes due to its comparatively slower pace and higher cost.
Next-Generation Sequencing
Next-Generation Sequencing (NGS) represents a significant advancement in sequencing technology, allowing for massive parallel sequencing. This means that millions of fragments can be sequenced simultaneously. NGS technologies have revolutionized genomics by providing a more scalable and efficient way to analyze entire genomes quickly and accurately.
The key characteristic of NGS is its ability to produce vast amounts of data in a relatively short time. This capability enables researchers to conduct large-scale studies and compare genetic variations across populations or even species. Additionally, NGS is useful for projects where the full genomic sequence is required, such as in genome-wide association studies.
Nevertheless, while NGS offers incredible benefits, it also comes with challenges. The data generated require extensive computational analysis, which can be a barrier for some labs without adequate resources. Furthermore, the initial setup for NGS can be costly, making it less accessible for smaller research facilities.
Bioinformatics Tools for Analysis
The analysis of DNA sequences has been greatly enhanced by bioinformatics tools. These tools are crucial for interpreting the vast amounts of data generated from sequencing technologies. They facilitate the alignment of sequences, identification of mutations, and prediction of protein structures. Popular bioinformatics platforms include BLAST for sequence alignment and a variety of software for genomic data visualization.
Applications of DNA to Amino Acid Conversion
The conversion of DNA sequences into amino acids is a cornerstone of molecular biology, presenting a wealth of applications across various fields. Understanding how DNA translates into proteins opens the door to innovations in biotechnology, medicine, and genetic engineering. Each application highlights the profound impact of genetic coding on health, agricultural advancements, and environmental sustainability. This section delves into specific elements of these applications, showcasing their benefits and the considerations necessary for responsible implementation.
Biotechnology and Medicine
Biotechnology leverages biological systems to develop products and technologies that significantly impact human health. The understanding of amino acid sequences derived from DNA enables researchers to engineer proteins with specific functions. These engineered proteins can be used in various ways:
- Therapeutic Proteins: Drugs like insulin and monoclonal antibodies are produced using recombinant DNA technology. For instance, insulin is synthesized by inserting the human insulin gene into bacteria, leading to large-scale production. This method offers a cost-effective and precise means of supplying essential medications.
- Vaccine Development: The rapid development of vaccines has been revolutionized through mRNA technology. By using mRNA that encodes viral proteins, the body can generate an immune response without introducing the virus itself. This approach has played a crucial role in response to pandemics, notably the COVID-19 crisis.
- Gene Therapy: Advancements in gene therapy aim to correct genetic disorders at the source. By understanding the protein encoded by a defective gene, scientists can introduce a healthy version of that gene to restore normal function. This technique is currently in use for several inherited conditions, showing promising results.
Despite these advantages, it is important to consider ethical implications and safety issues associated with biotechnological applications. Ongoing monitoring and regulation are essential to ensure these innovations do not lead to adverse effects.
Genetic Engineering
Genetic engineering, often called genetic modification, directly manipulates an organism's DNA to achieve desired traits. This field has seen significant growth, facilitated by the clear relationship between DNA sequences and their corresponding amino acid sequences. Key applications include:
- Crop Improvement: Genetic modification of plants enables the development of crops with enhanced traits. For example, genetically modified organisms can be engineered to be resistant to pests or harsh weather conditions, enhancing food security. Techniques such as CRISPR allow for precise edits to DNA, which can boost nutritional content or yield.
- Synthetic Biology: This emerging field combines biology with engineering principles to design and construct new biological parts. In synthetic biology, researchers can create entirely new proteins for specific tasks, such as biofuels or bioplastics, promoting sustainability and reducing reliance on fossil fuels.
- Animal Models for Research: Genetic engineering also allows the creation of animal models that mimic human diseases. These models are crucial for understanding disease mechanisms and developing new treatments, making research more applicable to human health.
In the context of genetic engineering, careful considerations about biodiversity, ecological impact, and long-term effects on ecosystems are also vital. Employing responsible practices will ensure that this powerful tool benefits society while minimizing unintended consequences.
"The manipulation of DNA sequences to generate specific protein products has transformed biotechnology and genetic engineering, leading to numerous advancements in health and agriculture."
Overall, the applications of DNA to amino acid conversion extend far into the realms of biotechnology and genetic engineering. By advancing our understanding and manipulating genetic material, there is immense potential to address pressing global challenges, as well as improve human health and food security.
Epilogue
The conclusion of this article highlights the significance of understanding the conversion of DNA sequences to amino acids. This process is fundamental to molecular biology and underpins all biological systems. Each segment of the article has aimed to clarify the intricate pathways by which genetic information is translated into functional proteins. It is essential to acknowledge that the knowledge gained here fosters a deeper appreciation for life at a molecular level.
Summary of Key Concepts
This section has laid out critical elements of the topic. Key concepts include:
- The relationship between DNA, mRNA, and amino acids.
- The nature of the genetic code and its codons.
- The process of transcription, including post-transcriptional modifications.
- Detailed steps of translation and the roles of ribosomes and tRNA.
- The implications of codon usage and its effect on protein synthesis.
- Understanding mutations and their potential consequences for protein function.
- The various experimental techniques utilized in sequence analysis, such as sequencing technologies and bioinformatics tools.
- Practical applications in biotechnology and genetic engineering that emerge from this foundational knowledge.
Through these topics, we have established a cohesive narrative that interlinks genetics, molecular biology, and their practical implications.
Future Directions in Research
Looking ahead, the future of research in DNA to amino acid conversion is promising. Key areas requiring further exploration include:
- Advances in sequencing technologies, which may enhance accuracy and reduce costs.
- The role of artificial intelligence in predicting protein structures from genetic information.
- The impact of epigenetics on gene expression and protein production.
- Continued investigation into the implications of gene editing technologies like CRISPR on the genetic code.
Research in these domains could yield significant advancements in how we understand genetic regulation, protein synthesis, and their applications in medicine and biotechnology. This foundational knowledge opens doors for innovation and the potential to solve complex biological challenges.