Exploring Transmitting Electron Microscopy Techniques
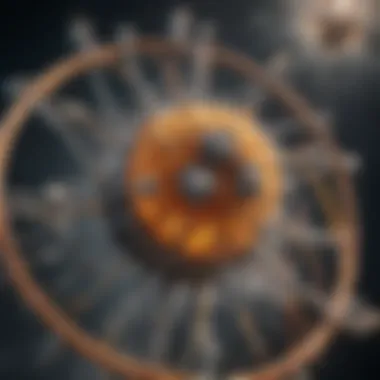
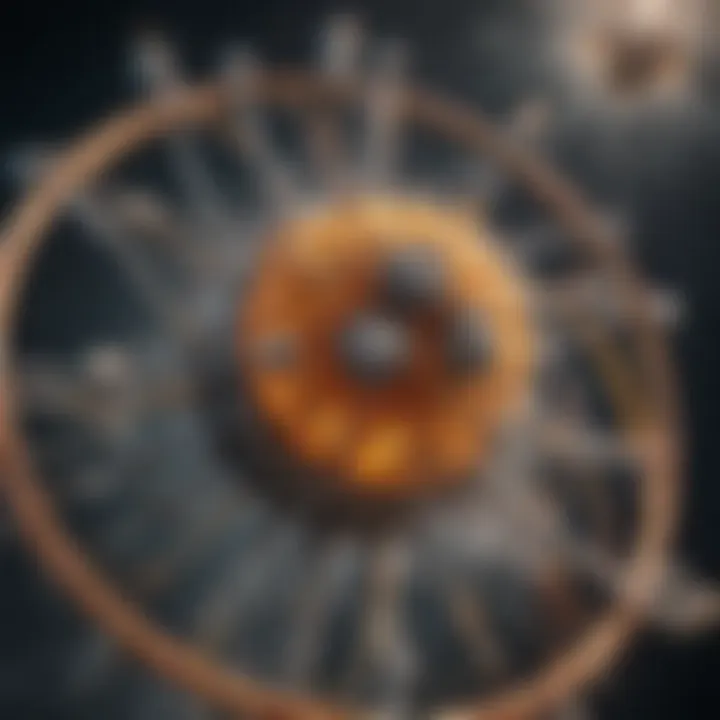
Intro
Transmitting electron microscopy (TEM) stands out as a remarkable approach for understanding materials at a level that traditional microscopy simply cannot reach. It opens up new vistas into the atomic structure of substances, enabling scientists to grasp their properties and behavior in unprecedented detail. In a world where materials innovation drives advancements in technology, medicine, and biology, the meticulous insights that TEM offers prove indispensable.
This article delves deep into the principles and methodologies that underpin TEM, providing clarity on how it works and why it holds such importance across various fields.
Recent Advances
In recent years, TEM has experienced considerable progress. This evolution not only enhances the technique itself but also broadens its application across multiple scientific disciplines. New findings are emerging almost weekly, with researchers across the globe pushing boundaries and breaking new ground. The latest insights in TEM provide a more nuanced understanding of materials, facilitating better designs and implementations in several domains.
Latest Discoveries
Some crucial discoveries that have surfaced include:
- Atomic-scale imaging of complex materials, allowing for the visualization of defects and impurities that were previously invisible to researchers.
- In situ TEM techniques, which permit the observation of materials in real time under operational conditions, shedding light on dynamic processes such as phase transitions and deformation.
- Enhanced sensitivity to chemical composition enables intricate studies of catalysts and nanostructures vital for energy applications.
These advancements not only reflect the ongoing refinement of TEM techniques but also spotlight their role in scientific research and industrial applications, making the technology more versatile and effective.
Technological Innovations
The evolution in hardware and software tools is substantial. Innovations in electron detectors, for example, contribute to better resolution and contrast, unveiling details with remarkable precision. Some notable advancements are:
- Direct detection cameras that offer higher sensitivity and faster frame rates, enabling clearer imaging of dynamic phenomena.
- Automated software that streamlines data analysis, making interpretations more accessible and less reliant on specialized training.
Such technological strides have played a pivotal role in making TEM more widely applied, ensuring it remains at the forefront of material characterization techniques.
Methodology
Understanding the methodology of TEM is fundamental to appreciating its capabilities. The procedure, while sophisticated, hinges on a series of steps that transform raw materials into images capturing their atomic structures.
Research Design
In the context of TEM, the research design can be broken down into a few key phases:
- Sample selection: Choosing the appropriate material, often dictated by the research question.
- Sample preparation: A pivotal task, this often includes thinning the sample to less than 100 nanometers, enhancing the likelihood of electron transmission.
- Data acquisition: Using various imaging modes, such as bright field or dark field, to gather necessary information based on the prepared sample.
Each phase is crucial and can significantly impact the quality and validity of the resulting data.
Data Collection Techniques
The way data is collected in TEM is intricate. Key techniques include:
- Electron diffraction: This provides crystallographic information about the materials, crucial for identifying phases or structures.
- Energy-dispersive X-ray spectroscopy (EDX): A component of TEM that allows for chemical analysis by detecting emitted X-rays.
Through these methods, TEM does not merely provide pictures; it articulates a detailed narrative of material composition and evolution on an atomic scale.
To sum it up, exploring TEM reveals not only its technical intricacies but also its profound importance in addressing key scientific questions. Whether it’s the latest discoveries or the overarching methodological rigors, each facet contributes to its standing as an essential tool in contemporary research.
"The power of TEM lies not merely in its ability to capture images, but in its capacity to open dialogues about the fundamental nature of materials we engage with every day."
In the ensuing sections, we will further probe the principles of TEM, alongside its applications across disciplines, ensuring a comprehensive understanding of this essential scientific tool.
Foreword to Transmitting Electron Microscopy
Transmitting electron microscopy (TEM) stands as a pivotal technique in the world of materials science and biological studies, granting researchers an acute view into the atomic landscape of matter. Its significance doesn't merely lie in its ability to magnify images but rather in the profound insights it provides about the structural organisation and properties of materials, making it an indispensable tool in scientific research. Understanding TEM is essential for various domains, from nanotechnology to medicine, as it allows for the analysis of materials at an unprecedented resolution.
Historical Context
The roots of transmitting electron microscopy can be traced back to the early 20th century, around the time when the concept of electron waves was introduced. The first practical demonstration came into play during the 1930s, when scientists such as Ernst Ruska and Max Knoll successfully constructed the first TEM. Their pioneering work was motivated by the limitations of optical microscopes, which struggle to resolve features beyond light’s wavelength.
Notably, the early advancements in this technology were closely linked with the development of electron sources and lenses, culminating in the fabrication of the first commercial TEM by the late 1940s. Over decades, researchers fine-tuned these instruments, enabling deeper explorations of various materials. Consequently, TEM machines became staples in laboratories worldwide, contributing insights across fields like metallurgy, nanotechnology, and biology.
Fundamental Concepts
To fully grasp the capabilities of TEM, it’s essential to understand several foundational principles. At its core, TEM operates on the principle of electron interaction with matter. An electron beam passes through a thin specimen, where various phenomena such as scattering, diffraction, and absorption occur.
Here are some core concepts to consider:
- Wavelength and Resolution: Electrons have much shorter wavelengths compared to visible light, allowing TEM to achieve resolutions down to the atomic level.
- Thin Film Requirement: Samples must be thin enough to ensure that electrons can transmit through them with reduced scattering. Typically, thicknesses of less than 100 nanometers are desirable.
- Image Formation: The resulting images are the echoes of how electrons are scattered by the specimen. Contrast in these images arises from variations in atomic number and thickness.
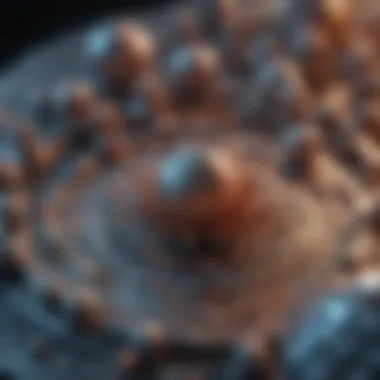
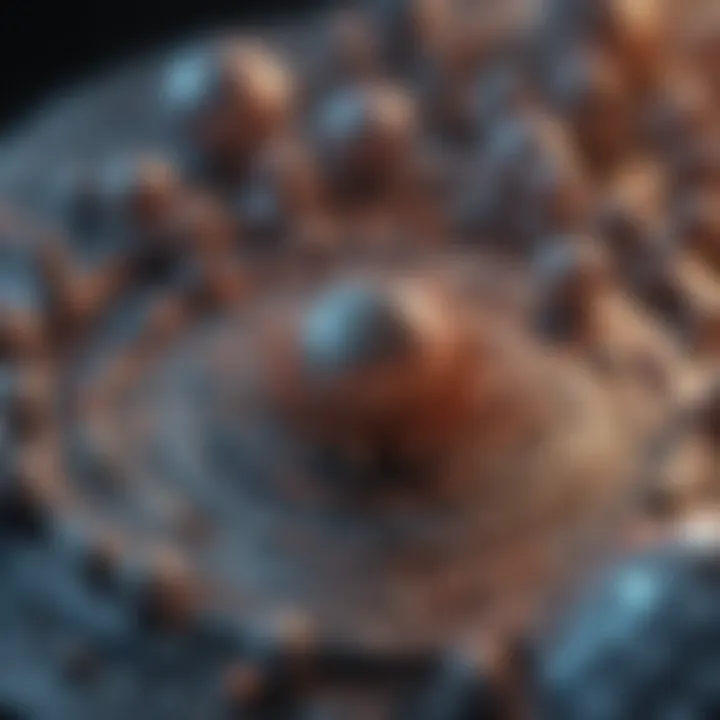
In essence, understanding these fundamental aspects opens the door to appreciating the intricate workings of TEM, setting the stage for its application in research and industry alike.
"Transmitting electron microscopy doesn't just show us images. It reveals the story hidden at the atomic level of all materials we encounter."
These insights position TEM as a critical technology in the realm of microscopic research, with applications that can change how we interact with and understand materials at the most granular level.
Core Principles of TEM
Understanding the core principles of Transmitting Electron Microscopy (TEM) is crucial as they form the bedrock of how this powerful imaging technique operates. Grasping these principles helps professionals in materials science, biology, and nanotechnology to employ TEM effectively in their research. Moreover, this compendium of knowledge aids in avoiding potential pitfalls during the application of this intricate technology.
Electron Beam Generation
At the heart of Transmission Electron Microscopy lies the electron beam generation process, which is pivotal for achieving high-resolution imaging. Unlike light in traditional optical microscopes, electrons have a shorter wavelength, enabling them to reveal structures at atomic resolutions.
The typical setup begins with a heated filament, often tungsten or LaB6, that emits electrons through thermionic emission. These electrons are then accelerated by high voltages, typically around 100 kV to 300 kV, creating a focused beam. This beam is essential as it determines the quality of the images obtained.
- Key Points About Electron Beam Generation:
- Voltage levels impact resolution and contrast. Higher voltages can provide greater depth of field but may introduce sample damage.
- The stability of the electron source influences the consistency of the imaging throughout the research.
- Better vacuum conditions reduce electron scattering, enhancing image clarity.
Interaction with Specimens
When the focused beam of electrons strikes the specimen, a series of interactions occur that are essential for image formation. Electrons can be scattered elastically or inelastically, depending on the atomic structure of the material. Elastic scattering is vital for preserving the original image information, while inelastic scattering can provide insights into the electronic structure of the atoms.
During this process, the specimen must be exceedingly thin, usually less than 100 nanometers, to allow electrons to travel through it without excessive scattering. This thinness leads to a unique challenge: the risk of damaging the sample due to electron beam intensity.
- Considerations in Specimen Interaction:
- Sample thickness should be optimized to balance between clear imaging and structural integrity.
- Different materials exhibit varied scattering behaviors, which is critical for interpreting the results.
Image Formation Mechanism
The image formation in TEM is a multi-step process that relies heavily on the interaction between the electron beam and the specimen. Once the electrons pass through the specimen, they must be accurately focused to create a coherent image. This is achieved using a set of electromagnetic lenses that manipulate the path of the electrons.
- Process Steps in Image Formation:
- Objective Lens: After passing through the specimen, electrons are captured by the objective lens, which magnifies the image.
- Intermediate Lens: Further magnification occurs with the intermediate lens, enhancing detail.
- Projector Lens: Finally, the projector lens directs the electrons onto a detector, leading to the formation of the final image.
Images produced can be further processed using advanced software to increase their clarity or highlight specific features.
Important Note: The skill in operating the TEM not only revolves around hardware but also the expertise in analyzing the resultant images to deduce qualitative and quantitative data about the specimen.
This foundational knowledge of electron beam generation, interaction with specimens, and image formation mechanisms is crucial for anyone looking to harness the power of TEM in their research. By comprehensively understanding these core principles, researchers can optimize their approach, ensuring that they capture the most informative and diagnostic images possible.
TEM Instrumentation
The art of transmitting electron microscopy (TEM) is firmly rooted not only in its principles but also in its instrumentation. The instruments used in TEM are not just mere machines; they are highly sophisticated systems that require a deep understanding to harness their full potential. This section focuses on the critical elements that make up a TEM system and highlights the benefits and considerations associated with it.
Basic Components of a TEM System
At the core of a TEM system, several fundamental components work together in harmony to produce high-resolution images at atomic scales. Understanding these components is essential for both transitions into operating the equipment and for interpreting the resulting data accurately.
- Electron Source: The heart of TEM lies in the electron source, typically a thermionic or field emission gun. This component generates a focused beam of electrons, which is crucial as the visualization of materials occurs at this microscopic level.
- Condenser Lenses: These lenses help shape and focus the electron beam onto the specimen. Depending on the type of analysis, adjusting these lenses changes the beam's properties, affecting the resolution and contrast of the resulting images.
- Specimen Holder: The specimen is placed in the holder, which can accommodate various sample types. It often includes mechanisms to control the sample's position and orientation, essential when dealing with nanostructures where even a slight misalignment can lead to significant errors in interpretation.
- Objective Lens: A crucial component that magnifies the image formed by the interaction of the electron beam with the specimen. The objective lens power influences the ultimate resolution achievable in the final image.
- Projector Lenses: Following image formation, projector lenses take the magnified image and project it onto a fluorescent screen or camera for observation and capture. This process bridges the microscopic world to something we can visualize and analyze.
- Detector Systems: These systems capture the electrons transmitted through the specimen. Different types of detectors, like charge-coupled devices (CCDs), are employed based on the intended outcome of the imaging process.
Advanced TEM Techniques
As research progresses, so does the technology and technique surrounding TEM. Advanced techniques enable researchers to enhance their data acquisition and analysis, contributing to the vivid details that TEM can unveil.
- High-Resolution TEM (HRTEM): This technique pushes the boundaries of resolution, allowing the visualization of atomic arrangements within materials. It involves carefully manipulating the electron beam and alignment to achieve clarity and detail that was once thought impossible.
- Scanning TEM (STEM): Unlike traditional TEM, this technique scans the electron beam across the sample. It provides real-time data and can yield both atomic resolution images and analytical information about the elemental composition of the sample.
- Electron Energy Loss Spectroscopy (EELS): EELS examines the energy lost by electrons as they pass through the sample, offering insights into the electronic structure, bonding, and even the chemical composition of materials at atomic dimensions.
- Cryo-TEM: Particularly significant for biological applications, cryo-TEM allows samples to be rapidly frozen to preserve their natural state. This technique opens a window into the processes of life itself by enabling the observation of biomolecules in their native environments without the artifacts associated with traditional sample preparation methods.
"The quality of any TEM study is dictated not just by the microscopy techniques employed but by the depth of understanding of the instrumentation itself."
For more detailed information on TEM components and applications, you might find resources like Wikipedia or articles on Britannica helpful.
Sample Preparation for TEM
Sample preparation is a crucial step in the process of utilizing Transmitting Electron Microscopy (TEM). It's not just about getting a sample under a microscope; it’s about providing the best possible representation of what you are trying to analyze. Properly prepared samples can mean the difference between a clear, informative image and one that is muddied by artifacts or distortions. Thus, understanding the nuances of this stage is fundamental to maximizing the potential of TEM as a characterization tool.
The benefits of meticulous sample preparation are manifold. Firstly, thin films or nanoscale samples significantly increase the transmission of electrons through the specimen, allowing for enhanced imaging contrast and resolution. Secondly, effective preparation helps in reducing surface contamination and structural artifacts, which can skew the results. As researchers dig deeper into the material characteristics, the clarity of the data becomes paramount.
Beyond image quality, prepared samples contribute to the accuracy of the analysis. Factors such as thickness, morphology, and crystallinity all play significant roles in how a material will interact with the electron beam. A well-prepared sample accommodates the electron beam’s path better, reducing scattering and allowing for more precise measurements. Consequently, the integrity of experimental results fundamentally hinges on the preparation techniques employed.
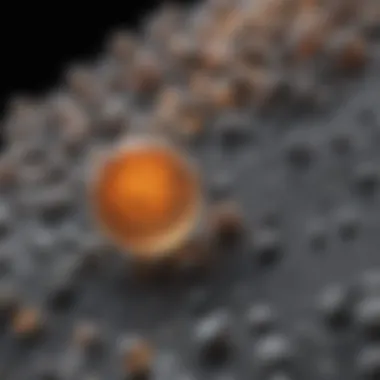
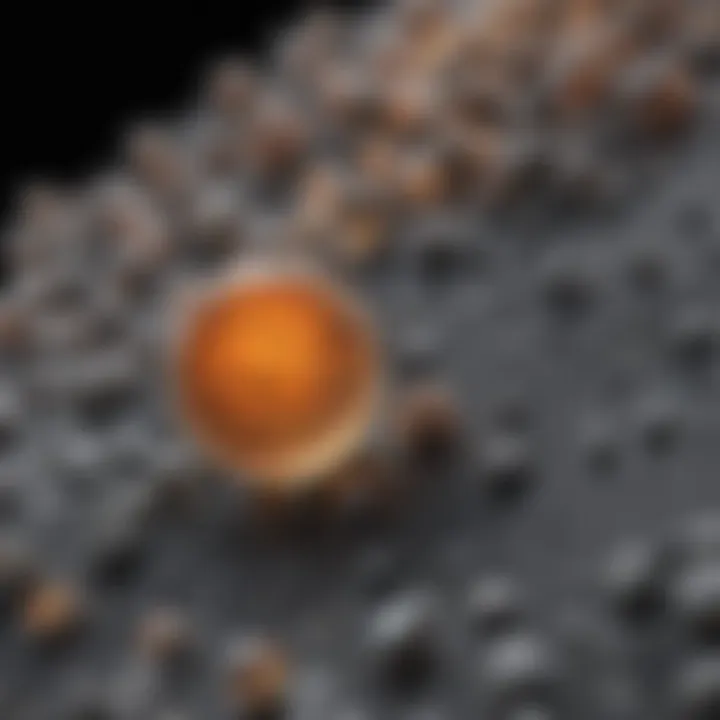
Techniques for Thin Film Preparation
Thin film preparation techniques, often considered an art and science in their own right, come in various forms. Each method carries with it unique advantages and limitations depending on the material and intended analysis. Some common methods include:
- Mechanical Cleaving: This simple yet effective technique involves cleaving the bulk sample to achieve a single-layer thickness. Ideal for materials like graphite, mechanical cleaving can produce reasonably thin sheets without the complexity of other methods.
- Ion Milling: Involves bombarding the sample surface with ions to achieve the desired thinness. This technique allows for better control over the sample’s final thickness but requires precision and often results in surface damage that must be mitigated.
- Sputtering: This technique deposits thin films by ejecting material from a target onto the substrate using ion bombardment. Its benefit lies in the ability to create uniform layers, but achieving ultra-thin layers for TEM analysis can be challenging.
- Cryl-Microtoming: Involves cutting thin slices (often below nanometer levels) using a diamond knife. This method is particularly advantageous for biological samples, and it retains the structural features better than other techniques.
Adapting the method to the material and analysis goal is essential. Finding the right technique can enhance the accuracy of results significantly, but it requires experience and skill to strike the right balance.
Challenges in Sample Preparation
No discussion about sample preparation would be complete without recognizing that it comes with its own set of challenges. Some of these include:
- Thickness Control: Achieving the optimal thickness is often easier said than done. Samples that are too thick will scatter electrons excessively, while those too thin risk breaking or becoming damaged, making control difficult.
- Contamination: Environmental factors can introduce contaminants during preparation, which will appear in the TEM images. This can be a significant PID (process-induced damage).
- Material Integrity: Some materials are sensitive to the electron beam and can change their properties during observation. This requires careful handling during preparation to preserve material characteristics.
- Complex Morphologies: Some samples have intricate structures that challenge traditional preparation techniques. Finding methods that respect the original morphology while achieving the needed thinness can be tricky.
Proper sample preparation is not just a step; it's foundational to the integrity of the data gathered through TEM. Even a minor flaw in preparation can lead to misleading results, impacting the entire research trajectory.
Understanding these challenges allows researchers to improve their sample preparation strategies, ultimately aiding in more reliable results. Proper planning and meticulous execution are key to overcoming these obstacles and achieving quality imaging from TEM.
Applications of TEM
Transmitting Electron Microscopy (TEM) stands as a vital tool across various scientific domains. The applications of TEM are not just a side note, but a pivotal component that showcases its significance in understanding materials at an atomic level. By unlocking detailed insights, TEM helps in shaping advancements in fields like materials science, biological studies, and nanotechnology. Each of these areas exemplifies the power of TEM, revealing not just the strengths of the technique, but also opening up discussions around its limitations and considerations that must be taken into account during research.
Materials Science
In materials science, TEM serves as a beacon for researchers keen on studying the intricate details of material structures. The ability to analyze metals, ceramics, polymers, and composites at a nanoscale is crucial for developing innovative materials that boast enhanced properties. For instance, through TEM, scientists can observe the arrangement of atoms and the defects that might affect a material’s performance.
Consider a scenario where researchers are aiming to improve the strength of a metal alloy. By using TEM, they can identify microstructural features such as grain boundaries and precipitates that influence mechanical properties. Understanding how these elements interact under different conditions can lead to tailored materials suited for specific applications, such as aerospace or biomedical implementations.
Some key points about materials science applications of TEM include:
- Characterization of nanostructures: TEM allows for the study of nanoparticles and their interactions, which are crucial for catalysis and drug delivery.
- Investigation of phase transitions: Researchers can observe how materials change phases at atomic levels, helping in the development of smarter materials.
- Assessment of damage mechanisms: Analyzing the failure of materials under stress provides insights for the creation of more durable options.
"TEM is not just about taking pictures; it’s about interpreting the atomic landscape that transforms the entire narrative of material development."
Biological Studies
The biological realm has equally embraced the capabilities of TEM. With its high-resolution imaging, researchers can delve into the realm of cellular structures and organelles. The application of TEM in biology unveils the complex architecture of cells, viruses, and tissues, offering insights into their functions and interactions.
For example, in virology, TEM plays a crucial role in characterizing virus morphology. By understanding the structure of a virus at such a small scale, researchers can identify potential targets for antiviral therapies. Also, the technique aids in observing cellular processes such as endocytosis and exocytosis, which are fundamental for understanding how cells communicate and interact with their environment.
Some notable contributions of TEM to biological studies include:
- Revealing cellular structures: Enables visualization of organelles, helping in understanding their functionality in health and disease.
- Studying sample interactions: Observing how cells respond to infections at a cellular level aids in drug development.
- Examining structural anomalies: Identifying pathological changes in tissues enables diagnostics for various diseases.
Nanotechnology
Nanotechnology thrives on the principles governed by TEM. At the nanoscale, the properties of materials can differ dramatically from their bulk counterparts. Thus, TEM becomes essential for the synthesis and characterization of nanomaterials. It provides not just images of nanostructures but also insights into how these materials behave under different conditions.
The crafting of nanomaterials often relies on precise measurements of their size, shape, and morphology, all of which are achievable through TEM. For instance, researchers can analyze quantum dots—tiny semiconductor particles that have unique optical properties used in display technologies. By assessing the size distribution of quantum dots, one can infer their electronic properties and thus tailor them for specific applications.
Key implications of TEM in nanotechnology include:
- Precision in characterization: Accurate measurement of size and shape critical for understanding the properties of nanomaterials.
- Development of novel applications: Insights from TEM pave the way for innovations, such as drug delivery systems where nanoparticles target specific tissues.
- Surface analysis: Helps understand how interactions at the nanoscale differ from conventional materials, guiding the design of better devices.
Data Analysis in TEM
Data analysis plays a pivotal role in Transmitting Electron Microscopy (TEM). Without it, the vast information captured through high-resolution images would remain a mystery, rendering the entire imaging process a mere exercise in observation. As such, understanding how to process and analyze the data collected during TEM studies is fundamental for unlocking the insights hidden within materials at the nanoscale.
- Importance of Data Analysis
In TEM, data analysis is crucial for transforming raw electron microscope images into meaningful interpretations. This stage is where intuition meets technology, encapsulating raw data into formats that enhance understanding. The quality of results derived from TEM is directly linked to the effectiveness of data analysis techniques employed. With the influx of data in modern microscopy, researchers must harness sophisticated analytical tools to sift through nuances. - Benefits to Research
The benefits of robust data analysis in TEM are multifold:Essentially, data analysis takes the microscope’s raw gaze and turns it into a lens of utility, filtering not just what is seen but interpreting its relevance.
- Enhanced Image Clarity: With the right image processing techniques, researchers can eliminate noise, sharpen details, and highlight critical features of the specimen.
- Quantifiable Insights: Data analysis enables quantification of parameters such as size, shape, and distribution of nanoparticles, helping to support scientific hypotheses with empirical evidence.
- Comparative Studies: By analyzing multiple samples in similar conditions, crucial correlations and differences can come to light, aiding in understanding material properties and behaviors.
Image Processing Techniques
When it comes to analyzing images captured by TEM, the importance of image processing techniques cannot be overstated. The journey begins with high-frequency signals from the electron beam, but without subsequent processing, these signals can remain unwieldy and complex.
Image processing techniques lie at the core of data clarity.* Here are some renowned techniques typically employed:
- Filtering: Various types of filtering techniques, such as Gaussian or median filtering, help reduce noise in the images. This is crucial for achieving cleaner and clearer visuals.
- Contrast Enhancement: Adjusting contrast can help highlight structural details that may not be evident in the original images. Techniques include histogram equalization, which redistributes image brightness.
- Segmentation: This breaks down an image into parts or segments, thus allowing for better analysis of specific structures within the sample.
By applying these techniques, researchers can create high-fidelity images that contain comprehensible data suitable for further interpretation.
Quantitative Analysis
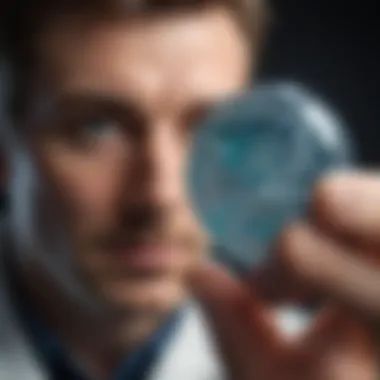
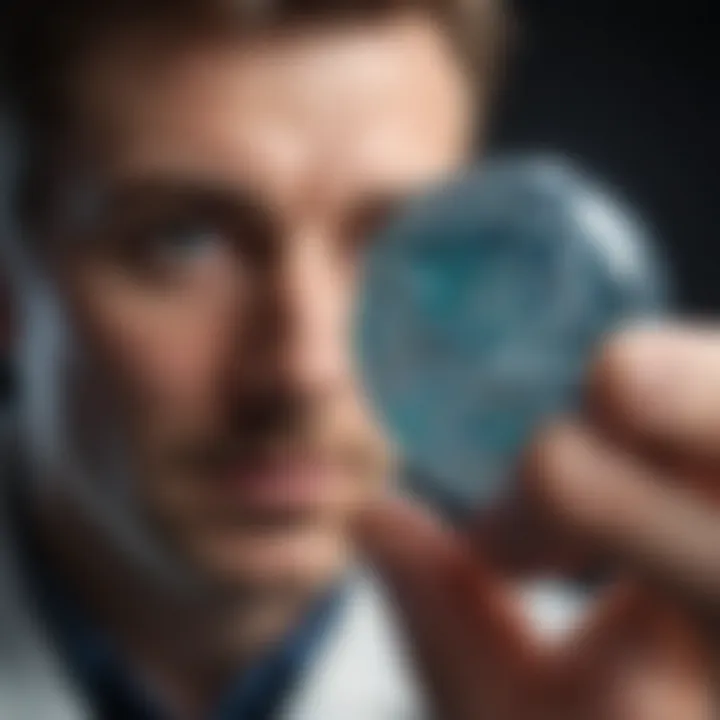
Quantitative analysis forms the backbone of data interpretation in TEM. This stage is where numbers speak louder than images, providing measurable evidence that supports theoretical frameworks.
A few key considerations in quantitative analysis include:
- Dimensional Measurements: Researchers often need to measure the thickness of materials, diameter of nanoparticles, or even the spacing of atomic layers. Precise measurements are critical for advancing materials science.
- Statistical Analysis: Conducting statistical analysis on image data allows researchers to mitigate the effects of sample variability. For instance, utilizing large datasets can yield statistically relevant conclusions regarding the properties of materials.
- Comparative Analysis: This technique helps in contrasting outcomes across different material samples or conditions, offering insights into how modifications affect performance.
Quantitative analysis in TEM isn’t just about counting and measuring. It's about drawing insightful conclusions, gaining predictive power over materials' behaviors, and ultimately guiding future research directions.
"In the world of TEM, data analysis isn't just an afterthought—it's the crucial thread that weaves observation into understanding."
Advantages and Limitations of TEM
Transmitting Electron Microscopy (TEM) holds a prominent place in the arsenal of techniques available for material characterization. Recognizing both its advantages and limitations is essential not only for students and researchers, but also for those educators and professionals keen on employing TEM in various applications. By understanding these aspects, one can better appreciate how this method fits into the larger scientific landscape.
Strengths of TEM
TEM stands out for a variety of reasons, making it invaluable in numerous fields. Here are some of its key strengths:
- High Resolution: One of the most significant advantages of TEM is its capacity to achieve atomic-scale resolution. This level of detail allows for incredible insights into crystalline structures and defects, which are crucial in materials science and nanotechnology.
- Versatile Contrast Mechanisms: With techniques like dark field and bright field imaging, TEM provides diverse imaging options, enabling users to visualize specimens in different ways. Different contrast techniques can highlight various structural features, giving a multi-faceted view of the sample.
- Elemental Analysis: TEM is not just about imaging; it can also perform spectroscopic techniques such as energy-dispersive X-ray spectroscopy (EDX). This allows researchers to simultaneously obtain information about the chemical composition of materials alongside morphological data.
- Dynamic Studies: In some cases, realtime studies under TEM can be executed, allowing scientists to observe changes in samples while they undergo transformation, such as phase changes or chemical reactions. This ability adds a whole new dimension to experiments.
"With TEM, you don't just look at materials; you can visualize the very building blocks they consist of"
Limitations and Challenges
While TEM has many strengths, it is not without its challenges and limitations:
- Complex Sample Preparation: Achieving quality results often requires highly skilled preparation methods. Specimens need to be extremely thin, generally below 100 nanometers, which can complicate the process and sometimes alter the sample's characteristics.
- Vacuum Conditions: The necessity of placing samples in a vacuum can be a drawback, especially for biological specimens that are sensitive to such environments. Changes in the state of the sample may occur, impacting results.
- Cost and Complexity: TEM instrumentation is expensive and requires significant maintenance. The complexity of operation means that proper training and expertise are required. This can limit accessibility for smaller laboratories or institutions.
- Limited Field of View: Due to the high magnification achievable, the field of view is often significantly narrow, meaning that obtaining a comprehensive overview of large samples may require multiple imaging sessions or adjustments to the setup.
By understanding these strengths and limitations, users of TEM can make informed decisions regarding its applications in various research endeavors. As one navigates the complex landscape of material characterization, TEM remains an indispensable tool, albeit one that demands respect for its particular complexities and nuances.
Future Trends in Transmitting Electron Microscopy
As the realm of nanotechnology and materials science expands, the landscape of Transmitting Electron Microscopy (TEM) continues to evolve. Keeping an eye on future trends in TEM not only sheds light on how the technique will be employed moving forward but also underscores its importance in tackling modern scientific challenges. The emerging technologies, analytical capabilities, and novel applications of TEM are pivotal in enhancing our understanding of materials at the atomic scale.
Emerging Technologies
The pace of innovation in TEM technology is remarkable. Newer electron sources, like field emission guns, can produce highly coherent and intense beams, improving image resolution. Furthermore, advancements in detectors are paving the way for faster and more accurate data collection. A notable example is the development of direct electron detectors, which can significantly enhance the quality of acquired images by reducing noise and improving signal-to-noise ratios.
The field is also witnessing an integration of artificial intelligence into TEM data processing. Machine learning algorithms are being employed to automate image analysis, potentially diminishing human error and speeding up workflows. This intersection of AI and microscopy heralds a new epoch in how data is interpreted. With real-time analysis becoming feasible, researchers may soon access insights that previously required extensive manual scrutiny.
Additionally, 3D electron tomography stands out as an advanced technique. It combines multiple 2D images from different angles to reconstruct a three-dimensional model of the specimen. This approach has paramount implications in fields like biology and materials science, enabling a more comprehensive understanding of structures and materials. Through this technology, researchers can visualize complex interactions at atomic levels, making it a game-changer in microscopy.
Emerging tools like 3D electron tomography not only enhance imagery but also open doors to novel research paths.
Potential Research Directions
As we glance into the future, several key research areas emerge as promising avenues for exploration within the domain of TEM. One such area is the study of 2D materials such as graphene and transition metal dichalcogenides. These exceptional materials possess unique properties that TEM can illuminate, leading to innovations in electronics and materials engineering.
In the biochemistry sector, TEM’s ability to visualize biomolecules at high resolutions stands to revolutionize drug design and molecular biology research. The capability to understand protein complexes at atomic detail can elucidate mechanisms of disease and drive the development of effective treatments.
Additionally, understanding thermal and mechanical behaviors on a nanoscale through in situ studies will become a focal point. Researchers are already investigating how materials respond under various conditions while being observed in real-time via TEM. This shift from mere observation to interactive study opens doors to previously unimaginable applications.
Moreover, with the quest for sustainability at the forefront of global concerns, there is growing interest in using TEM for catalysis research. Enhancing chemical reactions through a better understanding of catalytic processes on an atomic level offers opportunities for greener technologies.
In summary, the future of Transmitting Electron Microscopy is rich with potential. With continuous advancements in technology and methodologies, TEM is set to broaden its applicability across various scientific disciplines, paving the way for a deeper comprehension of the microscopic world.
Epilogue
In wrapping up our exploration of transmitting electron microscopy (TEM), it’s crucial to underline the various implications this technique holds for modern science. The collective insights from the detailed discussions highlight that TEM is not just a specialized tool but rather a fundamental component in the broader scientific toolkit.
Summation of Key Insights
One of the standout features of TEM is its ability to provide high-resolution images at the atomic level. This capability is invaluable across multiple domains of research. This isn't merely about seeing smaller things; it’s about understanding how the tiniest elements interact and form larger structures. Here are some key insights derived from the discussion:
- Precision in Imaging: TEM offers precision that is difficult to replicate with other imaging techniques. The clarity and detail it provides make it ideal for materials science and biological studies.
- Interdisciplinary Applications: From analyzing nanostructures to uncovering biological mechanisms, TEM provides versatile applications. It touches a variety of fields, contributing to advancements in nanotechnology, materials science, and biology.
- Impact on Research Quality: The insights gained through TEM allow researchers to draw more accurate conclusions. This enhances the overall quality of scientific research and promotes innovation.
"TEM bridges the gap between theoretical models and experimental realities, providing a clear window into the atomic landscape."
These factors collectively highlight how TEM serves as an integral part of the research heuristic, pushing the boundaries of what’s possible in scientific inquiry.
Implications for Future Research
Looking ahead, the trajectory of TEM promises significant developments. As technology offers newer opportunities, research is likely to morph in several ways:
- Emergence of Hybrid Techniques: The potential integration of TEM with other microscopy methods suggests enhanced capabilities. For instance, combining TEM with cryo-electron microscopy may pave the way for greater clarity in viewing biological macromolecules directly in their native state.
- Technological Advancements: Ongoing improvements in instrumentation, such as enhanced detectors and advanced imaging algorithms, will likely make TEM more accessible and user-friendly for researchers.
- Application in Real-Time Analysis: Future TEM approaches could involve real-time observation of dynamic processes in materials and biological systems. This leap could revolutionize fields, enabling immediate insights during experiments.
- Expanded Sample Types: Research will likely explore a broader spectrum of materials, including organic systems or even complex composite materials, thus extending the existing limitations of sample preparation and analysis.
In summary, as researchers continue to push the envelope, TEM will undoubtedly play a pivotal role in exploring the unknown, exemplifying how modern technology and scientific inquiry can harmonize to unveil the intricacies of the material world.