Comprehensive Guide to Mass Spectrometry Data Analysis
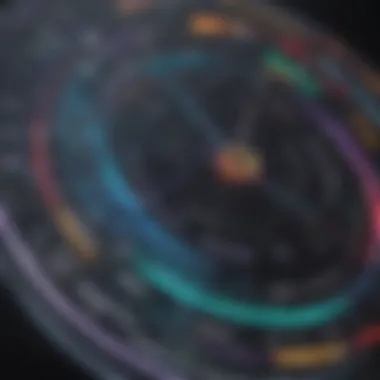
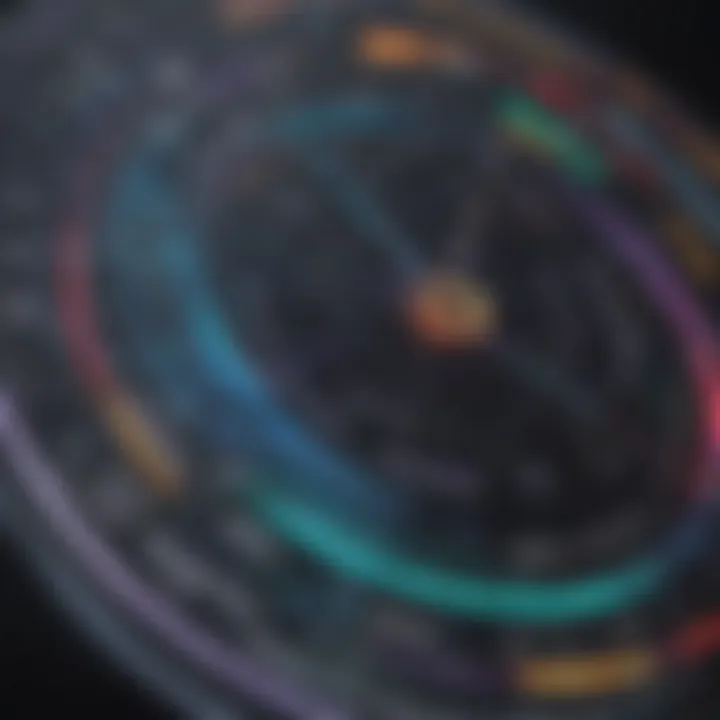
Intro
Mass spectrometry (MS) stands as a pillar in the realm of analytical chemistry, enabling researchers to identify compounds and elucidate molecular structures with remarkable precision. As the field burgeons, the need for adept data analysis has become ever more crucial. This section sets the stage by highlighting the significance of mastering mass spectrometry data analysis in today’s scientific landscape.
The complexity of interpreting MS data arises not only from the intricate machinery involved but also from the overwhelming amount of data generated during experiments. It's not just about running samples; it’s about understanding the underlying information those numbers represent. Success in this domain requires an exploration of both theoretical frameworks and practical methodologies—the very core of effective mass spectrometry data analysis.
Furthermore, as the lines between biology and chemistry blur, the importance of integrating mass spectrometry techniques into real-world applications cannot be overstated. For instance, in pharmaceutical research, the analysis of metabolites can make or break the development of new drugs. Similarly, in proteomics, identifying protein interactions can shed light on disease mechanisms. Thus, a well-rounded grasp of data analysis is essential—it’s like having the right map for a complex and winding path.
This tutorial will guide you through various components, from recent advances in the field to structured methodologies tailored for effective data analysis. Whether you are a fresh-faced student or a seasoned professional, this tutorial aims to enhance your skills and understanding of mass spectrometry data analysis.
"The journey of understanding mass spectrometry data is not just a path taken; it’s a voyage that intertwines curiosity with science."
Let's dive into the Recent Advances that are shaping the future of mass spectrometry.
Prelude to Mass Spectrometry
Mass spectrometry is more than just a flashy tool in the chemist's arsenal—it's a pivotal technique that allows researchers to dissect the molecular world. This section aims to lay the groundwork for understanding mass spectrometry by explaining its significance, its evolution over time, and the essential principles that govern how it operates. As we dive into this essential technique, we’ll explore not merely its scientific underpinnings but also its myriad applications across different fields of study.
History and Evolution
The roots of mass spectrometry can be traced back to the early 20th century, blooming from the minds of scientists seeking to analyze atomic particles more effectively. J.J. Thomson, in 1913, first paved the way by discovering the electron. His work eventually led to the development of the mass spectrometer in the 1920s. In those early days, the apparatus was cumbersome and relied on vacuum tubes and electrical fields, making it less accessible for widespread use.
Fast forward to the mid-20th century, when the rise of innovative technologies like the quadrupole mass filter significantly enhanced the ease and accuracy of using mass spectrometry. Over the decades, advancements such as the introduction of time-of-flight analyzers and mass spectrometry imaging have transformed the landscape, allowing for real-time analysis of compounds in diverse samples, from biological materials to environmental samples.
Principle of Operation
At its core, mass spectrometry revolves around principles of ionization and fragmentation. To distill a sample into its molecular components, molecules must first be ionized—this is done through several techniques, like electron impact and electrospray ionization. Once ionized, these molecules are accelerated into a mass analyzer, where they are separated based on their mass-to-charge ratio.
Here’s how it generally unfolds:
- Ionization: The sample’s molecules are transformed into ions.
- Acceleration: These ions are propelled into the mass analyzer.
- Separation: Ions are sorted based on their mass-to-charge ratio.
- Detection: The resulting spectra provide a detailed depiction of the sample's elemental and molecular composition.
A pivotal element of mass spectrometry is the ability to interpret the spectra generated during analysis. Each peak in the spectrum corresponds to an ion and can directly relate to a specific chemical species, depending on its mass and charge. This is where the art of mass spectrometry lies—knowing how to read these complex patterns to deduce information about the substances present in a sample.
Applications in Science
The versatility of mass spectrometry is perhaps its most striking feature. From pharmaceuticals to environmental science, its applicability is vast. In drug development, for instance, mass spectrometry aids in identifying and quantifying various drug compounds, ensuring their safety and efficacy. Similarly, in proteomics, researchers employ this technique to analyze proteins and their interactions, unraveling biological processes at a molecular level.
Among the key applications of mass spectrometry, one can highlight:
- Pharmaceutical Analysis: Ensures drug purity and formulation, identifying contaminants and degradation products.
- Clinical Diagnostics: Analyzes biological samples, facilitating early detection of diseases like cancer.
- Environmental Monitoring: Quantifies pollutants in air, water, and soil samples.
The implications of these applications are profound, as the technique not only enhances scientific understanding but also contributes to innovations in various sectors. The fusion of mass spectrometry with other analytical methods continues to push boundaries and drive forward scientific exploration.
"Mass spectrometry is like the scalpel of modern analytical chemistry—it cuts through the noise to provide clarity on the composition of matter."
As we progress into subsequent sections, we’ll take a closer look at the enumeration of components that make mass spectrometry what it is today, along with the practicalities of data acquisition and analysis.
Understanding Mass Spectrometry Components
Gaining a deep understanding of mass spectrometry components sets the stage for effective data analysis. Every element in the mass spectrometer, from the ionization techniques to the mass analyzers and detectors, plays a critical role in ensuring that accurate and meaningful data is generated. Understanding these components helps practitioners align their methodologies with the specific needs of their studies, leading to more reliable results. Key considerations include the selection of appropriate ionization methods based on sample types, the choice of mass analyzers that match resolution requirements, and the implementation of reliable detectors for capturing data effectively.
Ionization Techniques
Ionization is crucial because it transforms sample molecules into charged ions, which can then be analyzed based on their mass-to-charge ratio. Here’s a closer look at some common ionization techniques:
Electron Impact
Electron Impact (EI) is a widely adopted ionization method, especially in the realm of gas chromatography-mass spectrometry (GC-MS). The fundamental aspect of EI involves bombarding neutral gas-phase molecules with high-energy electrons, which leads to the ejection of electrons from the molecule, forming ions.
- Key Characteristic: A defining feature of Electron Impact is its ability to produce stable molecular ions, which can facilitate subsequent fragmentation patterns useful for identifying compounds.
- Benefits: Its popularity stems from the straightforward experimental setup and the reproducibility of results.
- Unique Feature: However, it does require volatile samples and can lead to excessive fragmentation, making it tricky for complex mixtures.
Electrospray Ionization
Electrospray Ionization (ESI) is favored in the analysis of large biomolecules, such as proteins and peptides. This technique involves the application of a high voltage to a liquid sample, producing charged droplets that evaporate, eventually forming ions.
- Key Characteristic: ESI is renowned for its softness, meaning it induces less fragmentation compared to EI, which is essential when preserving the integrity of larger molecules.
- Benefits: Its compatibility with liquid chromatography makes it a popular choice for biological applications.
- Unique Feature: On the downside, if analytes are not well dissolved, it can lead to poor sensitivity and inefficiencies.
Matrix-Assisted Laser Desorption Ionization
Matrix-Assisted Laser Desorption Ionization (MALDI) is another significant ionization method, particularly for biomolecules and polymers. It uses a laser to vaporize and ionize matrix material mixed with the analyte.
- Key Characteristic: A notable characteristic of MALDI is the minimal interaction between the charged matrix and analyte, which aids in preserving the analyte’s structure.
- Benefits: Its ability to analyze large molecules and solids grants it an edge when looking at complex biological samples.
- Unique Feature: However, sample preparation can be intricate, and not all samples are compatible, which might limit its applicability.
Mass Analyzers
Mass analyzers are integral to mass spectrometry, and they determine the mass-to-charge ratio of ions generated during the ionization process. Each type of mass analyzer has its unique mechanisms and specific applications.
Quadrupole
The Quadrupole mass analyzer is a staple in many mass spectrometers thanks to its versatility. It utilizes oscillating electric fields to filter ions based on their mass-to-charge ratio.
- Key Characteristic: Its compact design and easy integration into various setups make it a favorite among researchers.
- Benefits: It offers decent resolution while maintaining relatively high throughput, ideal for routine analyses.
- Unique Feature: Its main drawback is a limited mass range compared to other analyzers, like Time-of-Flight.
Time-of-Flight
The Time-of-Flight (ToF) analyzer measures the time ions take to travel a known distance, allowing for the calculation of their mass-to-charge ratios.
- Key Characteristic: ToF analyzers provide high sensitivity and resolution, especially beneficial in complex mixtures.
- Benefits: Their capability of analyzing a wide range of masses makes them optimal for various applications, from proteomics to metabolomics.
- Unique Feature: However, they often require careful calibration and can struggle with high concentrations of analytes.
Orbitrap
The Orbitrap employs an innovative electrostatic field to trap ions and measure their frequencies, translating those frequencies into mass information.
- Key Characteristic: Known for its exceptional resolution and mass accuracy, the Orbitrap stands out in complex analytical scenarios.
- Benefits: This high level of precision is crucial in fields such as proteomics and drug discovery, where accurate identification and quantification are paramount.
- Unique Feature: The complexity of its setup and maintenance can be a limitation for some laboratories striving for simplicity.
Detectors and Data Acquisition
Detectors play an essential role in the mass spectrometry data acquisition process. They convert the ionic signal generated from the mass analyzers into measurable data that can be analyzed.
- Detector Types: Various detectors, such as electron multipliers and ion counting detectors, differ in their mechanisms and sensitivity levels. The choice of detector can significantly affect the quality and reliability of data.
- Data Acquisition: Proper data acquisition ensures that all relevant information from the detected ions is recorded, facilitating comprehensive data analysis. Optimizing the speed and sensitivity of the acquisition process can enhance the overall efficiency of mass spectrometry analyses.
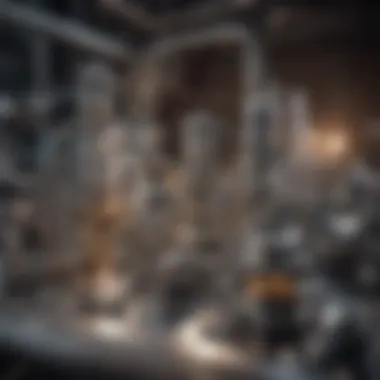
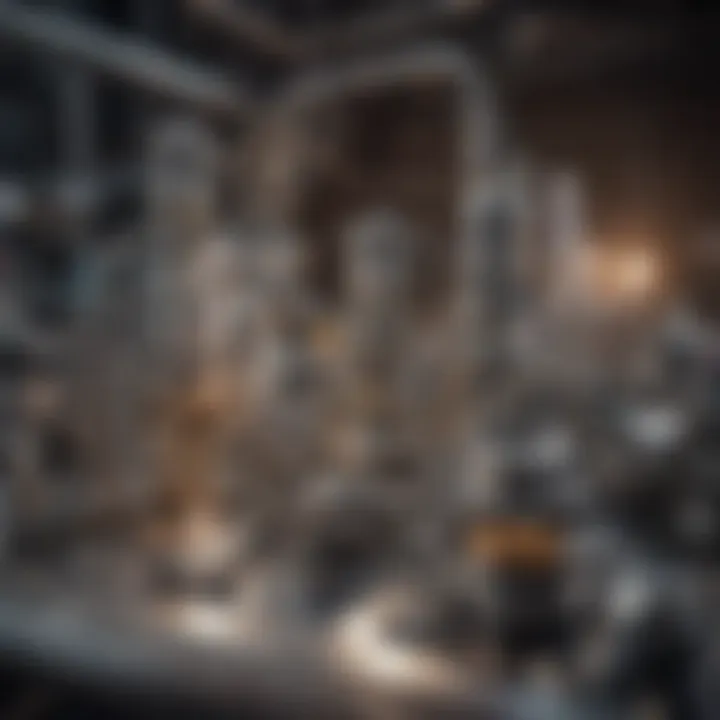
Understanding the distinct roles of each component in mass spectrometry allows researchers to select the optimal methods and instruments for their specific analytical needs.
Preparing Samples for Mass Spectrometry
The preparation of samples for mass spectrometry is a pivotal step in the analytical process, influencing the validity of the results obtained. Proper preparing techniques ensure that the samples are suitable for analysis, minimizing potential interferences and optimizing the data quality. A well-prepared sample can significantly improve the sensitivity and accuracy of the mass spectrometric analysis, leading to more reliable and reproducible results.
Sample Collection Techniques
Solid Samples
Solid samples come with their own set of unique considerations. They are often favored in various fields for their stability and simplicity of handling. One major characteristic of solid samples is their ability to retain their physical integrity during storage, which is not always the case for liquid or gas samples. This stability makes solid samples a beneficial choice for applications ranging from forensic analysis to food safety testing.
A key feature of solid samples is their potential for direct analysis without prior conversion to a liquid phase. This aspect allows for a cleaner analysis as it minimizes potential contamination during transfer processes. However, solid samples can also present certain disadvantages, particularly in the need for efficient ionization methods. For instance, techniques such as matrix-assisted laser desorption ionization are often required, adding complexity to the process.
Liquid Samples
Liquid samples excel in terms of versatility and ease of preparation. They can come from biological fluids, chemical formulations, or environmental sources, making them suitable for a wide range of applications. The primary characteristic of liquid samples is their ability to flow, which simplifies the process of ionization and ensures a uniform distribution across the mass spectrometer's inlet. This homogeneity can contribute significantly to achieving accurate readings.
The convenience of working with liquid samples makes them a popular choice for researchers. However, they also pose some challenges, including potential issues with evaporation or contamination, especially if not handled in a controlled environment. Additionally, sample viscosity may impact the efficiency of the mass spectrometry process.
Gas Samples
Gas samples are particularly notable for their application in detecting volatile compounds, which are often critical in fields like environmental monitoring and petrochemistry. The unique aspect of gas samples is their ability to disperse rapidly, allowing for quick analyses, which is especially advantageous in real-time monitoring scenarios.
Their key characteristic is the ease of ionization compared to solid and liquid samples, as gas samples can readily convert into ions within the mass spectrometer. This attribute positions gas samples as a beneficial choice for assessing air quality or investigating gas emissions. However, gas samples can be challenging to collect and maintain in a stable form for accurate measurements. Special collection devices may be required to prevent dilution or alteration of the sample composition during transfer.
Sample Preparation Methods
Dilution
Dilution is a fundamental method in preparing samples for mass spectrometry. This process is essential when dealing with concentrated samples, as it helps achieve the ideal concentration range for optimal ionization. The primary characteristic of dilution is its ability to reduce matrix effects, which can obscure the true signal from the analytes of interest. It’s a beneficial practice within the realm of mass spectrometry.
This method works by adjusting the concentration, allowing for better peak resolution in the spectra. However, excessive dilution may lead to loss of analyte sensitivity, which could adversely affect the overall results.
Filtration
Filtration is a critical preparation step designed to remove particulates or impurities from a sample. This process enhances the signal-to-noise ratio of the data, providing cleaner and more reliable results. The key characteristic of filtration lies in its ability to enhance sample purity, which is crucial for complex matrices.
Being thorough in this step is a beneficial choice that ensures only the analyte of interest is present during analysis. That said, filtration may also remove certain analytes along with unwanted substances, which could lead to under-reporting of specific compounds.
Derivatization
Derivatization is a sophisticated preparation method often employed to make analytes more detectable by enhancing their ionization properties. By chemically modifying compounds, this process can introduce functional groups that improve mass spectrometric performance. This method highlights the power of derivatization as a beneficial technique in mass spectrometry, particularly in enhancing sensitivity and selectivity.
However, it can also add complexity to the sample preparation workflow and may require specialized reagents and procedures, which could introduce variability and potential errors if not executed properly.
The importance of sample preparation in mass spectrometry cannot be overstated; proper techniques can be the difference between obtaining insightful data and inconclusive results.
In summary, the importance of preparing samples cannot be overstated in the context of mass spectrometry. Understanding the nuances of solid, liquid, and gas samples, along with various preparation methods like dilution, filtration, and derivatization, sets the stage for successful mass spectrometric analysis.
Data Acquisition in Mass Spectrometry
Data acquisition is a crucial phase in the mass spectrometry process. It acts as a bridge between the generation of ions and the eventual analysis of spectral data. By ensuring that data collected is both accurate and relevant, mass spectrometrists can greatly enhance the quality of the analysis. The importance of data acquisition lies not only in its immediate impact on the results but also in how it informs subsequent analysis and interpretation. Understanding this phase enables practitioners to address various factors that can influence the overall quality and reliability of mass spectrometry data.
It is important to consider the instrument calibration, as it sets the stage for data acquisition. Well-calibrated instruments can produce consistent and reproducible results, which are essential for valid comparisons over time. Inadequate calibration, on the other hand, may introduce errors that can lead to misinterpretations of the data.
Furthermore, choosing the right data collection strategy directly influences how the analysis unfolds. Different strategies cater to distinct analytical needs, allowing for flexibility in how data is captured. From a practical standpoint, such choices can affect everything from the sensitivity and specificity of the results to the speed and efficiency of the process. Knowing which approaches to apply and when becomes a skill that matures with practice.
"Data is the new oil" - a reminder of the value that high-quality data brings to any analysis, especially in mass spectrometry.
Instrument Calibration
Calibrating the instrument is fundamental to achieving accurate results in mass spectrometry. Calibration involves setting the mass scale and ensuring that the instrument is correctly measuring the mass-to-charge ratios of the ions produced. This step cannot be overstated; an uncalibrated or poorly calibrated instrument may lead to false results that could undermine the entire analysis.
A common approach involves the use of known standard compounds to check if the instrument's readings align with expected values. Regular calibration, especially before important or complex analyses, ensures reliability and enhances confidence in the results produced. For instance, if a mass spectrometer is off by even a small margin, it could easily misidentify critical compounds in a sample, which may have serious implications in fields such as pharmaceuticals or environmental science.
Data Collection Strategies
Effective data collection strategies can dramatically enhance the performance of mass spectrometry, shaping both the types of information obtained and their usefulness in resolving analytical challenges. Three primary strategies to consider are Full Scan, Selected Ion Monitoring, and Multiple Reaction Monitoring.
Full Scan
Full Scan is an approach where the instrument acquires data across a broad m/z range. This method allows the researcher to capture all the ions within that range, making it particularly useful when identifying unknown compounds. The characteristic of Full Scan is its comprehensive nature, as it does not limit the analysis to specific ions. This is beneficial as it allows for a more nuanced understanding of sample composition.
However, with the advantage of capturing a wide array of information comes a downside. The resulting dataset can be quite large and complex, requiring more processing power and time for analysis. Researchers often find themselves sifting through a large volume of data, which can complicate the identification of specific ions of interest if they are buried among many others.
Selected Ion Monitoring
In contrast, Selected Ion Monitoring (SIM) focuses on acquiring data from specific ions of interest. This targeted approach makes it a popular choice when monitoring the presence of particular compounds in a sample. The key characteristic of SIM is its heightened sensitivity to these selected ions, allowing for lower detection limits.
This method is particularly effective in scenarios where specific analytes are known, and researchers want to quantify them with precision. However, while it enhances sensitivity, it also narrows the focus, potentially overlooking relevant ions that might provide important context about the overall sample composition. Hence, in some situations, this strategy could lead to a less rich dataset.
Multiple Reaction Monitoring
Multiple Reaction Monitoring (MRM) takes the specificity of SIM a step further. Often used in quantitative measurements, MRM simultaneously monitors multiple target ions and their corresponding fragment ions. The main advantage here is that it can maximize sensitivity and selectivity, making it exceptionally powerful for applications like biomarker analysis or drug testing.
MRM’s unique feature lies in its ability to provide quantitative data on compounds even at very low concentrations. This is particularly useful in analyses that require stringent sensitivity criteria. However, the complexity of setting up MRM methods requires thorough preliminary research and validation of the chosen target ions, which can demand significant time and resources to establish correctly.
By understanding these data collection strategies and selecting the appropriate one, researchers can streamline their workflows and enhance the quality of mass spectrometry data significantly.
Data Analysis Techniques
Data analysis techniques play a vital role in the field of mass spectrometry, serving as the backbone of extracting meaningful insights from raw data. The importance of robust data analysis methods cannot be overstated, as they not only impact the accuracy of the results but can also define the overall success of research endeavors. The correct application of these techniques allows scientists to discern patterns, identify compounds, and quantify concentrations, making them indispensable in both academic and industrial settings.
Distinctly, data analysis can be bifurcated into basic and advanced techniques, each catering to specific needs within mass spectrometry studies. Basic data processing often includes straightforward methods that help in cleaning and standardizing data, whereas advanced techniques delve deeper into complex data structures and patterns, providing a more nuanced understanding of the samples under investigation.
This comprehensive look into data analysis techniques aims to arm students, researchers, and professionals with the necessary skills to navigate this intricate landscape. Incorporating elements such as data normalization and statistical validation enhances the overall quality of data interpretation, facilitating a more efficient workflow.
Basic Data Processing
Basic data processing refers to the initial steps taken to prepare mass spectrometry data for detailed analysis. This stage is critical as it helps in refining the data and eliminating any noise that could obscure significant findings. Common tasks in basic data processing include the following:
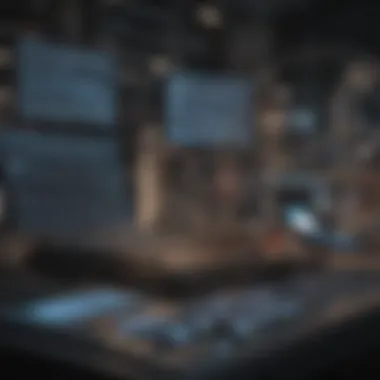
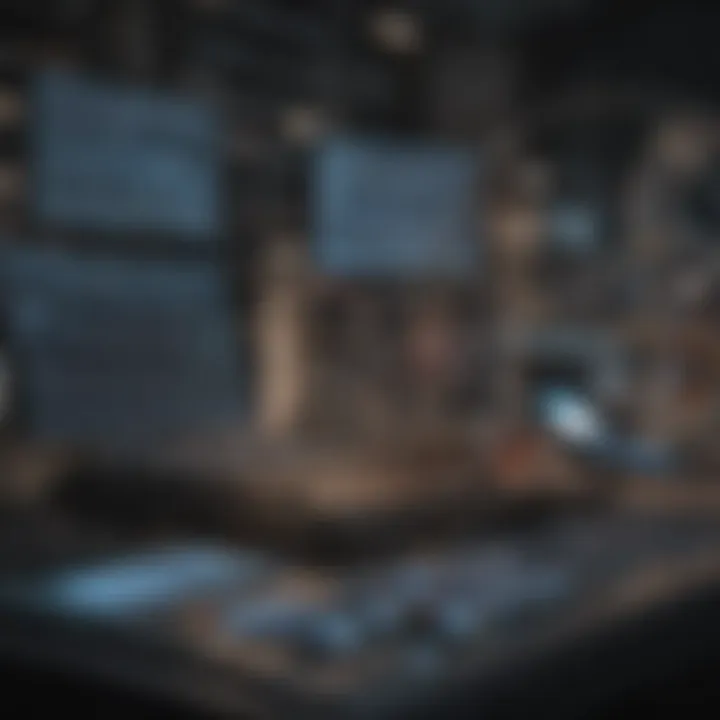
- Baseline Correction: Adjusting the baseline of the spectrum to remove any unwanted signals, allowing for a clearer interpretation of peaks.
- Peak Detection: Identifying key peaks within the spectrum that correspond to ions of interest.
- Data Normalization: Standardizing data sets to account for variations in sample preparation and instrument performance.
The quality of the basic processing steps directly affects the reliability of subsequent analyses. Neglecting this crucial stage can lead to flawed interpretations and misidentification of compounds. By focusing on these fundamental processes, researchers can avoid common pitfalls that may arise during later stages of analysis.
Advanced Data Processing
Advanced data processing encompasses more sophisticated analytical methodologies that are employed after basic processing is complete. Two prominent techniques within this category include deconvolution and isotope pattern analysis, both of which serve as instrumental tools in enhancing the understanding of mass spectrometry data.
Deconvolution
Deconvolution stands out as a significant method for resolving overlapping peaks in mass spectrometry data, effectively enhancing the clarity and interpretability of spectra. Through this technique, complex mixtures of compounds can be separated into individual components, allowing for a more precise analysis of their respective concentrations. The key characteristic of deconvolution lies in its ability to disentangle signals that may otherwise be indistinguishable in the raw data.
This technique is often regarded as beneficial because it significantly improves the accuracy of compound identification, especially in cases where multiple substances are present in similar mass ranges. A unique feature of deconvolution is its mathematical approach, which employs algorithms to model the spectral data.
However, it is not without its challenges. The process can be computationally intensive, requiring substantial analytical resources. Additionally, misinterpretations can occur if the existing algorithms are not tailored adequately to the specific characteristics of the samples being analyzed.
Isotope Pattern Analysis
Isotope pattern analysis plays a pivotal role in confirming compound identity through the examination of isotopic ratios. The key characteristic here is that many elements have multiple isotopes, and their relative abundances manifest as distinct clusters in mass spectra. This feature makes isotope pattern analysis a widely accepted and beneficial choice for those looking to validate molecular identities.
By analyzing the isotope distribution, researchers can infer the elemental composition of compounds and draw conclusions regarding their source and structure. The distinct advantage of this method is its ability to use naturally occurring isotopes to yield information that goes beyond simple mass readings.
However, isotope pattern analysis requires an understanding of isotopic distributions, which can be susceptible to variations depending on factors like instrument calibration and sample composition. Incorrect analysis could lead to erroneous confirmations, emphasizing the need for meticulous attention during this process.
In summary, mastering both basic and advanced data processing techniques equips practitioners with the tools necessary to excel in mass spectrometry analysis. The ongoing improvement in software tools and methodologies aims to bolster these processes, paving the way for more precise and informative outcomes in research.
Software Tools for Data Analysis
In the landscape of mass spectrometry, the ability to analyze data efficiently and accurately is crucial. This section delves into software tools for data analysis, shedding light on how these tools play a pivotal role in transforming raw spectral data into meaningful insights. With the rapid advancement in analytical chemistry, having the right software isn’t just a luxury; it’s a necessity that can significantly influence the outcomes of research.
Choosing the right software encompasses considering several factors:
- User Interface: Easy navigation is key, especially with complex data.
- Compatibility: It must work seamlessly with different mass spectrometry instruments.
- Support and Documentation: Good support can save users a lot of headaches.
- Cost: Balancing budget with capabilities is essential.
Efficient software tools enhance data quality and provide functionalities like data visualization, statistical analysis, and reporting, which can dramatically improve the researchers' productivity and the reliability of their findings.
Commercial Software Options
Among the various commercial software options available, there are several players that stand out, offering robust features tailored to both novice and advanced users. Companies like Thermo Fisher Scientific, Agilent Technologies, and SCIEX provide proprietary software designed specifically for their mass spectrometers.
These commercial offerings generally include:
- User-Friendly Interfaces: Designed for researchers at all skill levels, allowing quick access to essential functions.
- Advanced Data Processing Capabilities: Support for functionality like deconvolution and isotope pattern analysis.
- Customer Support and Training: Many commercial vendors offer workshops and support that can be invaluable to new users.
It's worth noting, though, that the cost associated with these software packages can be significant, making them less accessible for smaller labs or independent researchers. As such, understanding your needs and weighing the benefits against the cost is crucial before investing in these tools.
Open Source Tools
Open source software is gaining traction in the field of mass spectrometry, providing an alternative that can often compete with commercial options in terms of capabilities. Tools like OpenMS, MSFragger, and MaxQuant are noteworthy examples.
The advantages of using open source tools include:
- Cost Efficiency: Most open source software is free, which can make a huge difference for those on tight budgets.
- Flexibility and Customization: These tools often allow users to modify and adapt the software to suit their specific needs.
- Community Support: Open source projects usually boast active communities that contribute to their continued development and offer user support.
However, it’s important to approach open source with a measure of caution.
- Learning Curve: Some tools may require a deeper understanding of data analysis techniques and programming.
- Documentation Quality: Varies considerably, affecting how easy it is to find help when needed.
In sum, software tools for data analysis form the backbone of effective mass spectrometry, allowing researchers to draw conclusions from complex data sets. Balancing the options between commercial and open-source tools can greatly influence the efficiency and depth of analysis in any project.
Interpreting Mass Spectrometry Data
Interpreting mass spectrometry data is a cornerstone of analytical chemistry, acting like a compass, guiding researchers through the intricate paths of molecular identification and quantification. Understanding the results not only opens doors in research but also equips scientists to make informed decisions based on reliable data. Therefore, it’s key to grasp the significance of this topic and its impact on the broader landscape of scientific inquiry.
Understanding Spectra
Mass spectra serve as the blueprint of the analyzed sample, depicting the mass-to-charge ratio of ions present. The peaks in a spectrum represent the ionized components, where the height of each peak correlates to the relative abundance of the respective ion. A deep dive into how the peaks manifest can substantially impact interpretation. For example, the base peak is the highest point on the spectrum, representing the most intense signal, while the molecular ion peak hints at the weight of the entire molecule.
The appearance and resolution of the peaks depend on several factors, including ionization methods and mass analyzer sensitivity. Therefore, a thorough analysis begins by recognizing these aspects.
"Each peak has a tale to tell; silence can mislead the narrative of your data!"
Being aware of the factors influencing the spectra enables researchers to draw out valuable information. Keeping an eye on noise levels and potential artifact signals ensures that conclusions are drawn from genuine data rather than spurious findings.
Identifying Compounds
Identifying compounds through mass spectrometry involves a delicate balancing act between knowledge of mass spectra and analytical skills. It isn’t simply about matching peaks with a database; it's about evaluating fragmentation patterns and retention times. Various software tools and databases play a vital role here. Analysts often utilize libraries like NIST and other repositories to cross-reference and identify molecular structures based on mass spectral data.
Particular attention should be given to common fragmentation pathways that may be characteristic of specific classes of compounds. For instance, a hydroxyl group will show a distinct fragmentation pattern compared to an amino group. Here’s where having a robust understanding of organic chemistry becomes not just useful, but essential.
When employing multiple techniques, such as combining HPLC with mass spectrometry, the accuracy of identification can vastly improve. This multidimensional approach ensures that even the most elusive compounds can be discerned.
Quantitative Analysis
Quantitative analysis is the art of measuring the amount of a specific compound within a mixture, and mass spectrometry excels in this domain. The methodology relies heavily on the calibration of the instrument, where known standards are analyzed to create a reference curve. Typically, the linear dynamic range of the instrument is established, allowing for precise quantification across various concentrations.
When diving into quantification, consider not only the peak area but also the peak shape, as these can reveal additional insights into the sample makeup. The integration of peaks is crucial, as improper integration can lead to inaccurate estimations. Moreover, the use of isotopically labeled internal standards significantly boosts the reliability of quantification, compensation for ion suppression and variability during the analysis.
To sum it up, accurately interpreting mass spectrometry data entails understanding spectra, capabilities in compound identification, and applying best practices for quantitative measures. Approaching these analyses with diligence empowers researchers to unlock new avenues of discovery, ultimately advancing our understanding across various scientific fields.
Challenges in Mass Spectrometry Data Analysis
In the realm of mass spectrometry, understanding the data generated is as crucial as the technique itself. Gale winds of complexity often swirl around data interpretation. Without a solid grasp of the inherent challenges, practitioners risk missteps that can lead to inaccuracies, inefficiencies, and ultimately, compromised results. Here, we’ll focus on two pivotal challenges: matrix effects and ion suppression phenomena. These concepts not only impact data quality but also shed light on the intricate nature of mass spectrometry itself.
Matrix Effects
Matrix effects arise when other components present in a sample influence the detection of the target analytes, distorting the quantification and identification processes. This phenomenon is akin to trying to hear a conversation at a raucous party; the clamor of the matrix can drown out the voice of the target molecule.
Understanding Matrix Effects
Here are some critical dimensions of matrix effects:
- Nature of the Sample: The complexity of the matrix can vary tremendously—consider a biological sample like blood versus a simple chemical standard. The constituents of a biological sample can introduce variability that complicates analysis.
- Altered Ionization: The ionization efficiency may fluctuate when other molecules compete for ionization sites, changing the apparent concentration of the analyte.
- Quantification Accuracy: This effect can result in under- or overestimation of the target analyte concentrations, necessitating careful calibration and validation steps.
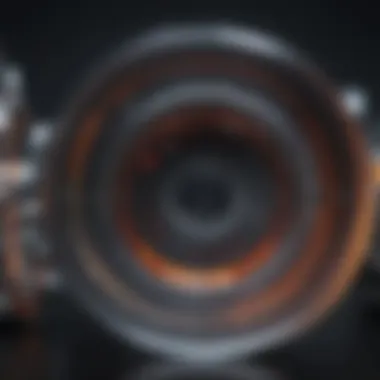
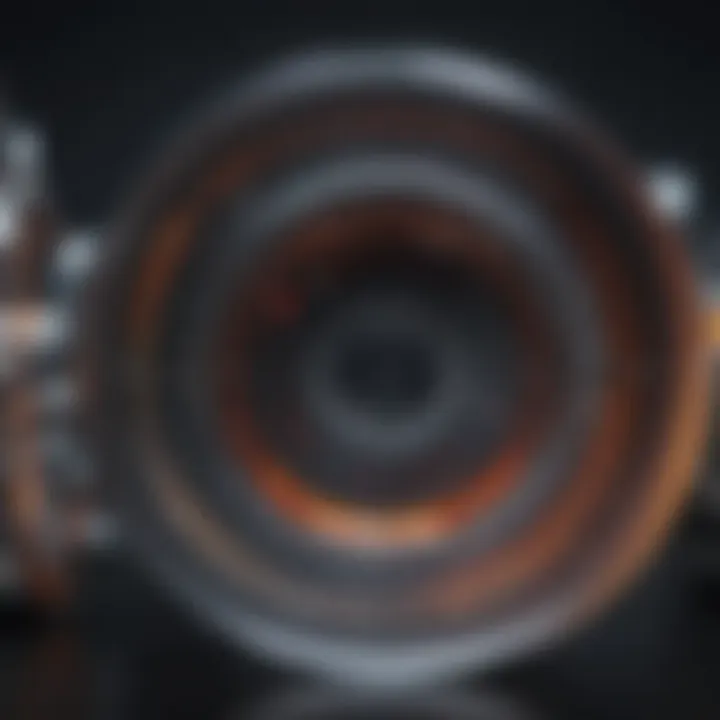
"Matrix effects can be a double-edged sword; they highlight the importance of meticulous sample preparation and method validation."
To mitigate the blow of matrix effects, researchers often invest time in method development. This might involve optimizing sample preparation protocols or employing internal standards to account for the variance introduced by the matrix.
Ion Suppression Phenomena
Ion suppression is a very specific subset within the landscape of matrix effects. It refers to the reduced response of an ion due to the presence of other ions in the mixture; this can significantly attenuate the signal of the target analyte. Imagine trying to decipher a delicate melody played on a violin while a brass band plays loudly in the background; the beautiful strains of the music may be nearly imperceptible.
Key Considerations on Ion Suppression
- Competitive Ionization: In many cases, more abundant ions can eclipse those of the analyte. For instance, when analyzing a peptide in a complex biological fluid, other more dominant ions can overshadow the signal from the peptide.
- Method Modification: To tackle this, mass spectrometrists may employ techniques such as adjusting ion source parameters, tweaking solvent ratios, or using different ionization methods.
- Data Validation: Continuous monitoring of ion suppression phenomena during data collection can inform necessary adjustments in real-time, enhancing the reliability of the results.
Real-world Applications of Mass Spectrometry
Mass spectrometry plays a pivotal role in various fields, extending far beyond the confines of laboratory walls. It acts as a key player in identifying compounds, unraveling complex biological systems, and ensuring environmental safety.
This section dives into practical applications that highlight the significance of mass spectrometry today. Understanding these applications is essential not just for researchers, but for anyone involved in scientific endeavors, as it showcases how theoretical knowledge transitions into real-world solutions.
Pharmaceutical Research
In the realm of pharmaceutical research, mass spectrometry serves as a cornerstone technique for drug development and analysis. The ability to accurately identify, quantify, and structure-react readily allows scientists to streamline the discovery of new drugs and ensure that existing medications are safe and effective.
For instance, during the drug formulation process, mass spectrometry helps in the assessment of stability, purity, and composition. It aids in verifying that none of the active ingredients are altered or degraded over time, an essential step to guarantee patient safety. Moreover, the capability of mass spectrometry to analyze metabolites and biomolecules contributes to pharmacokinetics, the study of how drugs move through the body.
Key advantages within pharmaceutical research include:
- Fast sample turnaround with high accuracy.
- Ability to analyze extremely small quantities of substances.
- Contributions to personalized medicine through metabolomics.
However, it's crucial to keep an eye on potential regulatory challenges. As pharmaceutical companies increasingly rely on mass spectrometry for comprehensive data analysis, ensuring compliance with stringent regulations can be daunting. Navigating the complexities of these requirements can be the difference between a drug making it to market or falling short.
Environmental Monitoring
Environmental science has also seen significant benefits from the application of mass spectrometry. This technique provides an efficient means of analyzing pollutants and understanding their impact on ecosystems and public health. It’s not just about detecting harmful substances; it’s about creating actionable insights that drive policy and protect our environment.
Mass spectrometry allows for the identification of trace levels of contaminants in air, water, and soil, helping scientists understand the dynamics of pollution. For example, when studying the effects of heavy metals on aquatic life, mass spectrometry can assess how quickly these metals accumulate in organisms, which informs conservation efforts and regulatory actions.
Key benefits of using mass spectrometry for environmental monitoring include:
- High sensitivity for detecting low concentrations of pollutants.
- Capability to provide detailed molecular information on complex mixtures.
- Insights into the sources and pathways of environmental pollutants.
While its advantages are considerable, practitioners must also be aware of the limitations. Sample preparation can be time-consuming and may introduce artifacts. Plus, interpretation of mass spectra from environmental samples requires experience and could be prone to errors if not managed correctly.
"Mass spectrometry has not just made waves in specific industries; it has created ripples across sciences, influencing everything from drug discovery to environmental stewardship."
Building expertise in understanding these real-world applications of mass spectrometry can significantly enhance the competencies of students, researchers, and professionals alike. The benefits herein underscore why mass spectrometry is an indispensable tool in various research and industry settings, showcasing the transition from theory to practical, impactful application.
Best Practices for Effective Analysis
Effective analysis in mass spectrometry is not just about having advanced equipment or mastering the theoretical aspects of the method; it’s also about employing best practices throughout the testing process. These practices ensure reliable and reproducible results, which is critical for making informed decisions based on the data obtained. With the continuous advancements in technology and increasing complexity of samples, understanding and implementing these best practices is more crucial than ever.
In this chapter, we will delve into two main aspects of best practices: quality control measures and documenting results. Both are significant to safeguard the integrity of the data while enabling seamless communication among researchers and analysts.
Quality Control Measures
Quality control (QC) measures serve as the backbone of robust mass spectrometry analysis. These measures help in identifying potential flaws at various stages of the experimental process. When it comes to QC, it is vital to recognize that even minor errors can exponentially affect results, leading to misinterpretations or erroneous conclusions. Here are several important quality control strategies to implement:
- Calibration: Regular calibration of instruments is essential. By ensuring that the equipment provides accurate readings, you reduce uncertainties in your data.
- Internal Standards: Use of internal standards can help account for variability during the analysis. By comparing quantification against known standards, you can achieve greater precision in your measurements.
- Replicates: It's wise to run replicates of samples, as this helps to identify variations within the analyses. Statistically valid conclusions require robust datasets that truly reflect the sample being studied.
- Blanks and Controls: Running blanks and control samples can aid in detecting any contamination or carryover effects that might compromise results. These controls provide a baseline to interpret the extent of detected signals.
- Routine Maintenance: Regular upkeep of the analytical equipment ensures its optimal functioning. Neglected machines can lead to drift in measurements, resulting in inaccurate data.
Implementing stringent quality control measures can substantially elevate the reliability of mass spectrometry analyses.
Documenting Results
The process of documenting results cannot be overstated. Proper documentation is not just about recording findings; it’s about creating a transparent framework that can be revisited or scrutinized in future studies. Such thoroughness offers several advantages. Here are a few key practices for documenting results effectively:
- Consistent Format: Use a consistent, well-structured format for documenting results. Standardized formats improve accessibility and comprehension by others who might refer to your work.
- Detailed Annotations: Additional notes regarding instrument settings, sample preparations, or any observations during analysis can be valuable. These annotations can inform others about potential idiosyncrasies associated with experiments.
- Data Storage: Utilize reliable data storage solutions to safeguard results. Digital databases, along with adequate backup systems, promote data longevity and accessibility.
- Open Access Sharing: Whenever possible, consider sharing raw data and metadata in open access platforms. Not only does this promote transparency, but it can also encourage collaboration and validation from other researchers.
In summary, both quality control measures and meticulous documentation are vital for effective mass spectrometry analysis. They enable researchers to ensure integrity in their analyses and foster collaboration within the scientific community.
Future Trends in Mass Spectrometry
As we dive into the future of mass spectrometry, it becomes clear that this field is on the brink of significant breakthroughs. Understanding these emerging trends is not just for academic curiosity; it holds practical implications for a breadth of applications ranging from drug discovery to environmental safety. The advancements in technology, mass spectrometry methodologies, and data analysis are essential discussions for researchers and practitioners alike.
Emerging Technologies
One cannot overstate the impact of technology in mass spectrometry. Trends to watch include:
- Miniaturization: Recent years have seen significant strides towards making mass spectrometers smaller without compromising performance. Portable devices are emerging for on-site analysis. This is particularly useful in fields like environmental monitoring, where immediate results can inform actions.
- Hybrid Mass Spectrometers: The combination of various mass spectrometry techniques leads to improved sensitivity and resolution. For instance, integrating Orbitrap and time-of-flight technologies can enhance the detection of complex compounds in biological samples.
- Ion Mobility Spectrometry (IMS): An evolving technique that separates ions based on their size and shape. It is gaining traction as a powerful tool in proteomics and the analysis of larger biomolecules, where traditional methods might fall short. Since IMS can provide additional structural information, it opens new doors for understanding biomolecular interactions.
These advancements not only broaden the scope of mass spectrometry applications but also increase the richness of data available for analysis. As instruments become more sophisticated and user-friendly, expect to see a surge of new researchers entering the field.
"The future of mass spectrometry is bright, with continuing innovations poised to revolutionize our analytical capabilities."
Integration with Other Analytical Techniques
As the complexity of samples increases in many research areas, the integration of mass spectrometry with other analytical techniques is becoming crucial. Some notable integrations include:
- Chromatography: Pairing mass spectrometry with techniques like High-Performance Liquid Chromatography (HPLC) or Gas Chromatography (GC) allows for better separation and identification of components before mass analysis. The combination often results in enhanced sensitivity and specificity, essential for quantifying trace levels of substances.
- Nuclear Magnetic Resonance (NMR): Though fundamentally different, the pairing of NMR with mass spectrometry provides comprehensive data about molecular structure and concentration. This combination is invaluable in small molecule discovery and development, particularly in the pharmaceutical sector.
- Imaging Mass Spectrometry: This newer approach combines mass spectrometry with imaging technology. It facilitates the visualization of the spatial distribution of compounds within a sample, which can aid in the study of tissues or complex mixtures. For example, researchers can better understand drug distribution in tissues by visualizing how compounds are distributed.
In summary, the intersection of mass spectrometry with other analytical frameworks not only enhances our capacity to analyze complex samples but also leads to more insightful results that unravel the intricacies of molecular interactions and functions. As we advance, this synergy will likely define the next generation of scientific discoveries.
Finale and Summary
In the realm of mass spectrometry data analysis, the conclusion and summary section holds considerable importance. It acts as a final bridge linking all the discussions and concepts presented throughout the article, providing a roadmap for both novices and seasoned professionals. The crux of synthesizing information is not just about reiterating previous points but also about framing them in a way that emphasizes their relevance in real-world applications. This is crucial, as the field of mass spectrometry continues to evolve at a rapid pace, influenced by technological advancements and new methodologies.
A well-crafted conclusion serves several purposes:
- Encapsulates Key Themes: It provides a compact overview of significant elements covered in the article, such as the principles of operation, sample preparation techniques, and the intricacies of data interpretation. By doing so, readers can grasp the essence without sifting through dense content.
- Highlights Practical Applications: The real beauty of mass spectrometry lies in its applicability across various fields. The conclusion reiterates how the discussed techniques contribute to advancements in pharmaceuticals, environmental science, and beyond. It touches upon the potential implications of effective data analysis in addressing complex scientific questions.
- Guides Future Exploreation: By summarizing the challenges and future trends, the conclusion encourages readers to continue their studies. It invites them to explore how emerging technologies and integration with other analytical techniques can revolutionize the field.
In short, this section permeates the tutorial with a sense of closure while inspiring further inquiry into the robust world of mass spectrometry.
Key Takeaways
- Understanding the importance of data analysis in mass spectrometry is crucial for proper application in various scientific fields.
- Effective sample preparation and data acquisition strategies significantly influence the quality of results obtained.
- Familiarity with different software and advanced processing techniques is necessary for accurate interpretation and identification of compounds.
- Continuous learning and adaptation to new technologies enhance analytical capabilities and the breadth of applications.
Further Reading
For those looking to deepen their knowledge in mass spectrometry and related techniques, consider exploring the following resources:
- Wikipedia: Mass Spectrometry
- Britannica: Mass Spectrometry Overview
- Discussions and insights can be found on forums such as Reddit Mass Spectrometry.
- Engage with professional communities or relevant groups on Facebook to network with experts and peers.