The Microfluidic Computer: A New Era in Computing
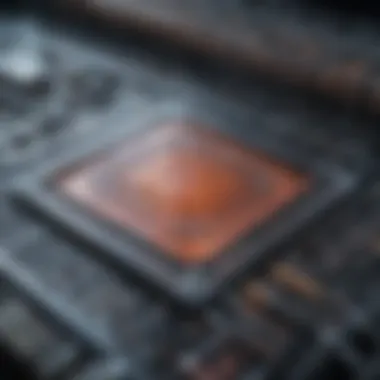
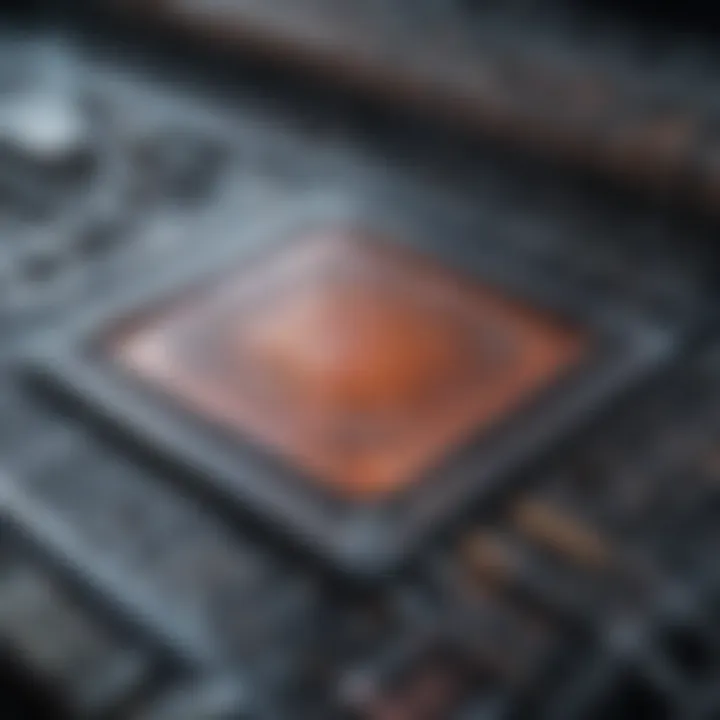
Intro
The exploration of microfluidic computers emerges from the intersection of fluid dynamics and computational science. This field holds significant promise, influencing various sectors such as biomedicine, environmental monitoring, and data processing. As we delve into this exciting domain, it is essential to understand the foundational principles that underpin microfluidic systems and their integration with computing technologies.
Microfluidics refers to the manipulation of fluids at a very small scale, typically in channels with dimensions ranging from tens of micrometers to millimeters. This technology enables precise control over fluid movement and interactions, permitting complex chemical reactions and biological processes to occur in a controlled environment. The adaptability of microfluidics allows these systems to be utilized not only in laboratories but also in portable diagnostic devices and environmental sensors.
In recent years, the development of microfluidic computers has gained attention. The advances in material science and design methodologies contribute to the feasibility of integrating computational functions into microfluidic platforms. By employing fluidic algorithms, such systems can perform computations traditionally handled by electronic computers. This transition is not merely technological; it is a redefinition of how computation can happen using physical phenomena.
As we continue through this article, we will underscore recent advances in microfluidic computing, focusing on innovations and research that shed light on its future potential. Subsequent sections will also address methodologies in research design and data collection techniques necessary for understanding this nexus of science and technology. Through this exploration, our aim is to provide a detailed and insightful framework for appreciating the transformative impact of microfluidic computers.
Preamble to Microfluidics
Microfluidics is a pivotal technology that fundamentally alters the way we approach computation and data analysis. The significance of microfluidics extends beyond traditional engineering into various domains such as biomedicine, environmental monitoring, and material science. By employing minuscule amounts of fluids in microchannels, this field facilitates processes that can be both rapid and precise, enabling innovative applications not previously possible with larger-scale systems.
Through this article, we will explore the technologies and methodologies in microfluidics that underpin the development of microfluidic computers. Such systems integrate fluid dynamics with computation, creating platforms that can perform data processing tasks using physical mechanisms rather than conventional electronic ones. This shift is particularly relevant in scenarios where speed and efficiency are crucial.
Understanding microfluidics is important for multiple reasons:
- Precision: Manipulating small volumes of liquids allows for meticulous control over reactions and processes.
- Cost-Effectiveness: Microfluidic devices typically require less reagent, which translates to lower operating costs.
- Versatility: This technology can be applied in diverse areas, making it valuable across scientific disciplines.
By examining the definition and historical background of microfluidics, we will gain a comprehensive understanding of its evolution and the foundational principles that inform current practices.
Definition and Scope
Microfluidics refers to the manipulation of fluids at the microscale. This field encapsulates the study and design of systems that handle liquid volumes typically in the range of nanoliters to microliters. These systems often utilize channels that are only a few micrometers wide, permitting remarkable control over fluid behavior. The scope of microfluidics extends to multiple applications, ranging from diagnostic devices to lab-on-a-chip technologies, revolutionizing fields like healthcare and environmental science.
Key aspects of microfluidics include:
- Fluid Dynamics: The principles governing fluids under micro-environments, where laminar flow predominates, are central to microfluidic designs.
- Integration: The ability to combine multiple functionalities into compact devices is a hallmark of microfluidic technology.
- Automation: Many systems are capable of being fully automated, improving efficiency.
Historical Context
The roots of microfluidics can be traced back to the mid-20th century, alongside advancements in microfabrication techniques. Initial research was concentrated on understanding the behavior of fluids in confined geometries. Over the years, innovations in materials and manufacturing processes have propelled microfluidics from theoretical exploration into practical applications.
The progress in this field was further catalyzed by the growing demand for rapid diagnostic techniques during the late 1980s. Researchers began utilizing soft lithography, a microfabrication technology that significantly reduced the cost and time associated with developing microfluidic devices. As the technology matured, it expanded beyond medical applications and into environmental monitoring and materials science.
Fundamentals of Microfluidic Systems
Microfluidics has emerged as a critical frontier in diverse fields ranging from biomedicine to environmental science. Understanding the fundamentals of microfluidic systems is essential for grasping how they redefine computational paradigms. Microfluidic technology manipulates small volumes of fluids, typically in the microliter to picoliter range. This enables high throughput and efficient analysis, which are crucial in applications such as drug development and environmental monitoring. Moreover, the ability to precise control fluid movements at microscopic scales allows for innovations in diagnostics and real-time monitoring.
Fluid Dynamics in Microchannels
Fluid dynamics in microchannels involves the study of how fluids flow through constrained geometries. At this scale, the dominance of viscous forces over inertial forces shifts the traditional understanding of fluid behavior. The low Reynolds numbers typically encountered in microfluidics denote laminar flow, leading to predictable and orderly fluid movement.
One key benefit of this flow regime is precise control over mixing and reaction processes. This can be especially advantageous in biomedical applications, such as in the development of assays for diagnostics. For instance, the mixing of reagents can be fine-tuned to enhance reaction rates, thereby increasing detection sensitivity.
To understand this concept, consider the following elements involved in fluid dynamics in microchannels:
- Laminar Flow: Smooth, orderly fluid motion, which allows for reliable outcomes.
- Diffusion: The mixing of substances occurs via molecular diffusion, which is relatively slow in larger volumes but can be manipulated through channel design in microsystems.
- Surface Tension Effects: This plays a pivotal role in shaping fluid behavior in microfluidic devices, affecting droplet formation and stability.
Physical Principles Governing Microfluidics
Physical principles governing microfluidics encompass fluid mechanics, thermodynamics, and transport phenomena.
The fundamental equations at play include the Navier-Stokes equations, which describe how the velocity field of a fluid evolves over time under applied forces. Other principles like capillarity are significant in microfluidic systems, where the interaction between liquid and solid interfaces can lead to unique behavior such as self-pumping in microchannels.
This section builds upon pivotal physical concepts:
- Pressure-Driven Flow: Ensures that fluids can be efficiently transported throughout microchannels by applying differential pressure.
- Electrokinetic Flow: Allows manipulation of liquids through electric fields, showcasing versatility in operations.
- Heat Transfer Mechanisms: Important in cases where thermal reaction conditions need to be controlled, preventing undesired temperature fluctuations.
In summary, grasping fluid dynamics and essential physical principles is crucial for anyone involved in microfluidic research or applications. The interplay between these fundamentals lays the groundwork for innovative computing platforms that take advantage of fluid behavior to process information efficiently and reliably.
"Microfluidics stands at the intersection of fluid dynamics and computational science, paving new pathways for research and technology across various sectors."
Design and Fabrication Techniques
The design and fabrication techniques for microfluidic devices are critical for the realization of microfluidic computing. These processes are at the heart of how microfluidics integrates with computational capabilities. The efficiency of data throughput, computational accuracy, and overall functionality of these systems heavily depend on design choices and the materials selected during fabrication. Key aspects include the scalability of production, the precision of the microstructures, and the porosity of the materials used. Understanding these elements offers insights into optimizing microfluidic devices for various applications.
Materials for Microfluidic Devices
The selection of materials is fundamental for the effective functioning of microfluidic devices. Various materials are utilized, including polymers, glass, and silicon. Each material has its unique properties that can significantly impact device performance.
- Polymers: Polydimethylsiloxane (PDMS) is frequently used due to its optical transparency, ease of fabrication, and compatibility with biological assays. Other polymers, like PMMA, are also gaining attention due to their rigidity and chemical resistance.
- Glass: Glass microfluidic devices are valued for their high compatibility with various biological and chemical processes. Glass channels can provide low adsorption of proteins and other biomolecules, which is essential for many applications in biomedicine.
- Silicon: Often utilized in the production of electronic components, silicon offers excellent structural properties and can be integrated easily with electronic circuits. This aspect is particularly important in the development of hybrid microfluidic systems that require immediate computational capabilities.
Choosing the right material involves balancing cost, availability, and specific operational requirements of the intended application.
Microfabrication Processes
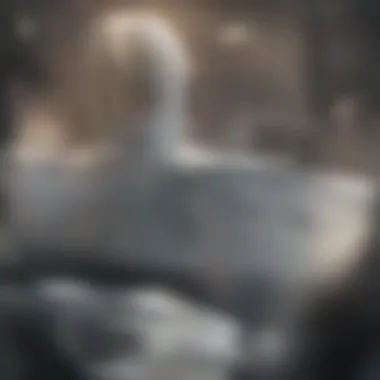
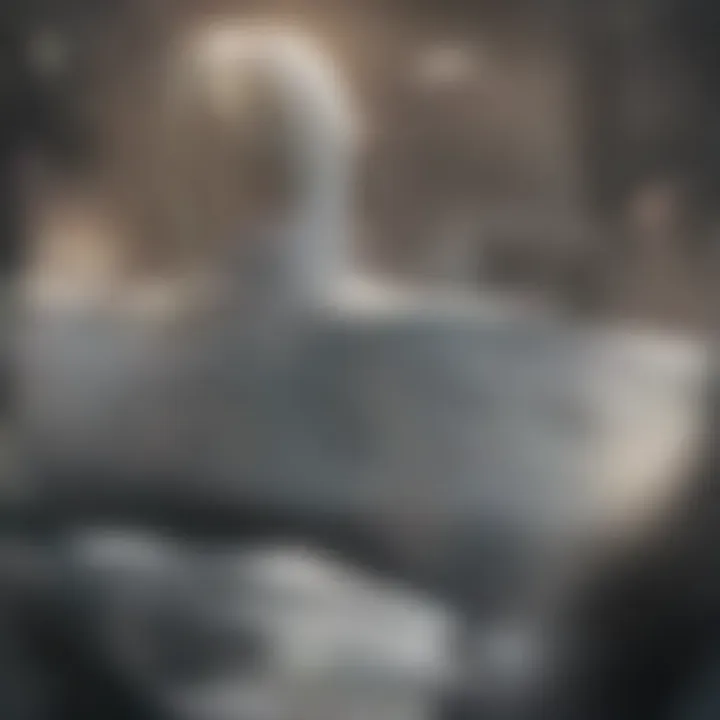
Microfabrication is the process that allows for the creation of microfluidic devices. This method utilizes a variety of technologies to fabricate the structures that control fluid flow on the microscopic scale.
Key processes include:
- Photolithography: This technique is often the first step in creating microfluidic devices. It uses light to transfer geometric patterns from a photomask to a light-sensitive chemical on a substrate, defining the channel layout.
- Soft Lithography: Particularly popular for PDMS-based devices, soft lithography involves creating a mold from a photolithographed master. This mold can be reused to cast multiple devices, thus enabling batch production.
- Etching Techniques: These involve removing material to create channel designs. Wet etching uses liquid chemicals, while dry etching employs plasma technologies. Both techniques require careful control of process parameters to ensure the desired channel depth and material quality.
- Bonding: After fabrication, bonding methods are essential for sealing microfluidic channels. Common techniques include plasma bonding and thermal bonding, which create strong, precise seals essential for operational integrity.
The development of these fabrication processes significantly influences the precision, efficiency, and scalability of microfluidic devices.
Overall, design and fabrication techniques are intertwined, forming the foundation for successful microfluidic computing systems. By improving both areas, researchers can harness the full potential of microfluidic technologies, leading to innovative applications across numerous fields.
Integration of Computing in Microfluidics
The integration of computing within microfluidic systems marks a significant turning point in technology. Combining two seemingly distinct fieldsβfluid dynamics and computational analysisβhas led to innovative approaches to data processing. This section delves into the particular elements that underpin this integration, along with its benefits and essential considerations that researchers and developers must keep in mind.
This fusion is not merely about embedding computing functions within a fluidic system; it represents a paradigm shift in how data is handled and analyzed at a microscopic scale. Microfluidic systems, through their intricate control of microscale fluids, provide an environment where data can be processed in real-time. This capability allows for unprecedented speed and precision when handling complex information. Moreover, the application of microfluidic circuitry can lead to substantial reductions in sample volumes and reagent usage, which is particularly beneficial in various settings including medical and environmental applications.
Some specific elements of this integration include:
- Real-time Processing: Enabling immediate responses to changes in fluidic environments.
- High Throughput: Processing a multitude of samples or data points simultaneously, which is crucial for applications like drug testing.
- Miniaturization: Compact designs that reduce space requirements, making systems more portable and affordable.
- Automation: Streamlining workflows that enhance reproducibility and reliability of results.
As the technology advances, considerations surrounding the integration of computing in microfluidics become increasingly important. Researchers must address issues such as:
- Technical Compatibility: Ensuring that computational systems can effectively interact with fluidic components.
- Data Security: Safeguarding sensitive information generated through medical or environmental monitoring applications.
- Scalability: Developing systems that can be adapted for both small-scale experiments and larger industrial applications.
"The integration of computing within microfluidic systems allows for innovative approaches in how data is handled at microscopic scales, fostering advancements in various fields."
In summary, integrating computing in microfluidic systems offers a host of possibilities that professionals across various sectors are beginning to explore. As this integration continues to evolve, it will offer deeper insights into complex data, ultimately redefining computational paradigms in ways we have yet to fully imagine.
Microfluidic Circuitry
Microfluidic circuitry serves as the backbone of the computational aspect of microfluidics. These circuits are designed to manipulate fluids in a controlled manner while simultaneously processing data. The design of microfluidic circuits involves creating channels, valves, and reactant mixing zones at a microscale level. This enables precise control over the fluid movement, which is essential for successfully processing samples and analyzing results accurately.
Several types of circuitry have emerged, including:
- Analog Circuits: These circuits allow for continuous control of fluid movement and mixing, essential for applications requiring graded responses.
- Digital Circuits: Adaptable systems that incorporate boolean logic, facilitating the execution of complex decision-making processes based on fluid properties.
- Hybrid Circuits: A combination of both analog and digital components, enabling versatile applications in both simple and complex analyses.
Advancing technology may usher in more sophisticated circuit designs, enhancing efficiency and versatility.
Data Processing Mechanisms
Data processing mechanisms are critical in transforming raw data collected from microfluidic experiments into valuable insights. These mechanisms can take various forms, depending on the complexity and requirements of the application. Commonly utilized data processing techniques include:
- Signal Processing: Enhances the clarity of readings and facilitates real-time analysis of data captured by sensors.
- Machine Learning Algorithms: Employed to improve predictive modeling and automate assessments, making systems more efficient.
- Statistical Analysis Tools: Used to interpret results, identify patterns, and derive significant conclusions from experimental data.
Using these data processing mechanisms helps to transform information into actionable knowledge, driving advancements in fields such as biomedicine and environmental monitoring. The ongoing development in this area, informed by emerging computational theories, promises to enhance the efficacy of data analysis in microfluidic contexts.
Applications in Biomedicine
The intersection of microfluidic technology and biomedicine presents significant advancements that can enhance medical practices and research. Microfluidic computers offer precise control over fluid dynamics at a microscale level, enabling real-time data processing and analysis. This capability is particularly pertinent for medical diagnostics and drug development, two areas where timely and accurate information is critical.
Medical Diagnostics
Microfluidic systems have revolutionized medical diagnostics by providing faster and more reliable testing methods. Traditional diagnostic procedures often require large samples and lengthy processing times. In contrast, microfluidic devices can perform tests on microliter volumes of fluids, significantly reducing the amount of biological material needed.
- Speed and Efficiency: These devices enable rapid analysis, essential for acute care settings where time is of the essence. Many microfluidic systems can deliver results in minutes, a substantial improvement over conventional methods.
- Point-of-Care Testing: The miniaturization of diagnostic tools facilitates point-of-care testing, allowing healthcare providers to conduct tests at the patientβs location. This shift not only decreases wait times but also enhances patient satisfaction and improves clinical outcomes.
- Multiplexing: Microfluidic platforms can analyze multiple targets simultaneously. This feature is advantageous for diseases that require the assessment of different biomarkers to confirm a diagnosis.
A prime example of microfluidic application in diagnostics is the development of lab-on-a-chip devices. These compact units integrate various laboratory functions into a single platform. This integration contributes to a decrease in costs and logistical challenges associated with transporting samples to centralized laboratories.
"Microfluidic devices hold the potential to not just change diagnostic processes but to save lives through timely intervention and personalized treatment options."
Drug Screening and Development
The impact of microfluidic technology extends deeply into drug screening and development. Pharmaceutical research often requires testing thousands of compounds over a long duration. Microfluidic systems accelerate this process, ensuring that potential treatments can be identified more efficiently.
- High-Throughput Screening: Microfluidic devices allow researchers to screen numerous samples in parallel while using minimal reagents. This efficiency can significantly cut down both time and cost associated with drug discovery.
- In Vitro Models: The ability to create microenvironments that mimic physiological conditions is crucial for effective drug testing. Microfluidics supports the development of tumor-on-a-chip models, which can provide insights into drug responses of specific cancer cell types.
- Personalized Medicine: The integration of microfluidics into drug development paves the way for personalized medicine. This approach tailors treatment based on individual patient profiles, enhancing the efficacy of drugs while minimizing adverse effects.
The benefits of microfluidic technology in drug screening cannot be overstated. With the continuous evolution of this field, the expectation is that microfluidics will not only expedite drug discovery but also lead to more targeted therapies in biomedicine.
Environmental Monitoring and Analysis
Environmental monitoring plays a crucial role in assessing the state of ecosystems, public health, and safety. Microfluidic computers offer new capabilities for real-time analysis with improved sensitivity and efficiency. The small scale of microfluidic systems allows for precise control and manipulation of fluids, which is essential in detecting environmental contaminants. These systems can provide immediate feedback, addressing the need for timely responses to environmental hazards.
The integration of computing into microfluidics leads to faster and more reliable data collection. Traditional analytical methods often require time-consuming sample preparation and analysis, while microfluidic technologies can streamline these processes significantly. This speed is vital in situations where rapid assessment is necessary.
Key benefits of microfluidic environmental monitoring include:
- High sensitivity: Microfluidics can detect low concentrations of pollutants, which is essential for effective monitoring.
- Reduced sample volume: Smaller sample sizes are not only economical but also minimize waste.
- Portability: Many microfluidic devices can be made compact, allowing for field testing and real-time monitoring outside the laboratory setting.
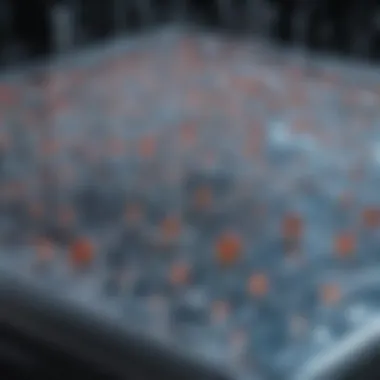
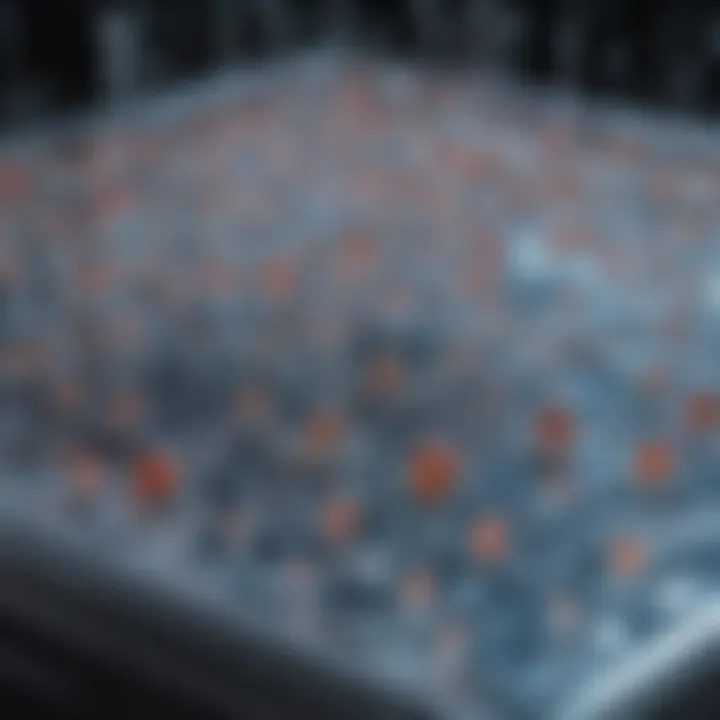
Considering the pressing environmental challenges we face today, these technological advancements are quite valuable. They allow for more informed decision-making regarding environmental management and policies.
Detection of Contaminants
Microfluidic systems are proving to be powerful tools for the detection of contaminants in various environments, including water, soil, and air. These devices utilize the principles of microfluidics to analyze tiny volumes of samples, offering rapid and sensitive detection capabilities. Since pollutants often exist in low concentrations, it is essential that detection methods are both precise and sensitive.
Some important methods for contaminant detection using microfluidics include:
- Immunoassays: Leveraging antibody-antigen interactions to identify specific contaminants.
- Electrochemical sensors: Using electrodes built into microfluidic channels to detect changes in chemical properties due to pollutant presence.
- Fluorescent assays: Employing labeled molecules that emit light when they interact with certain contaminants.
These methods contribute to a more thorough understanding of environmental health. The rise of microfluidic technologies enables on-site analysis, facilitating quicker interventions by regulatory bodies.
Real-Time Water Quality Assessment
Real-time water quality assessment is vital for ensuring public health, especially as freshwater sources become increasingly threatened. Microfluidic computers can perform continuous monitoring of key water parameters such as pH, turbidity, and contaminant levels. The ability to evaluate these factors in real time can substantially improve our response to water quality issues.
Microfluidic devices designed for water analysis can:
- Provide immediate data: Users gain insights quickly, allowing for faster decision-making.
- Integrate multiple analyses: Different parameters can be tested simultaneously, offering a comprehensive view of water quality.
- Connect to remote systems: With IoT integration, data can be sent to centralized databases for broader analysis and monitoring.
Real-time assessment through microfluidic technologies may transform how communities perceive and manage their water resources. By continuously monitoring environmental conditions, we can better predict and respond to harmful effects on both human populations and ecosystems.
Challenges in Microfluidic Computing
The domain of microfluidic computing comes with significant challenges that can hinder its advancement and practical application. Understanding these challenges is critical for researchers, engineers, and professionals interested in developing more efficient systems.
Microfluidic systems, while promising, face a variety of obstacles related to their technical limitations and scalability. These issues must be addressed to fully realize the potential of microfluidic technologies in various fields, including biomedicine, environmental monitoring, and analytical chemistry.
Technical Limitations
Technical limitations represent some of the most pressing challenges in microfluidic computing. These limitations can influence the overall performance and reliability of the systems. Key factors include:
- Size Constraints: Traditional computing elements are not easily reduced to the micro scale required for microfluidics. This leads to difficulties in integrating microprocessors and other components efficiently.
- Fluidic Control: Achieving precise control over fluid flow is critical in microfluidic systems. However, variations in fluid properties and channel geometry can cause unpredictable behavior, affecting computational outcomes.
- Material Challenges: Selecting appropriate materials for microfluidic devices is a complex task. Materials must be compatible with fluid dynamics while also withstanding chemical interactions, temperature variations, and potential contamination.
- Signal Processing: The need for effective signal processing presents additional challenges. Systems must be able to interpret and process data accurately from various sensors, a task that involves sophisticated algorithms and hardware integration.
It is crucial for researchers to innovate and find solutions to these technical barriers to enhance the efficacy of microfluidic computing systems.
Scalability Issues
Scalability is another significant challenge that researchers encounter when working with microfluidic systems. It pertains to the ability to produce and replicate devices in a cost-effective and efficient manner. Areas of concern include:
- Production Costs: Microfabrication techniques can be expensive, and scaling these processes for large production runs may not be economically viable. This limits the accessibility of these technologies, especially for startups and smaller organizations.
- Design Complexity: As systems become more complex, the design process can become convoluted. Designing a robust and scalable system that remains efficient at larger scales is a balancing act that requires in-depth computational modeling and simulation.
- Integration with Existing Systems: Another challenge lies in integrating microfluidic computers with existing technologies. Seamless integration is necessary to ensure that microfluidic devices can function harmoniously with current infrastructures, which may require significant modifications.
Addressing scalability issues is vital for the broader application of microfluidic computers. Without resolving these concerns, the widespread adoption of this technology remains uncertain.
In summary, while microfluidic computing holds great promise, overcoming technical limitations and scalability issues is essential for harnessing its full potential. Ongoing research efforts must focus on innovative solutions that can enhance the capabilities and accessibility of these advanced systems.
Current Research Trends
Importance of Current Research Trends
In the evolving landscape of microfluidic computers, research trends are critical for growth and innovation. These trends not only highlight the latest advancements but also point towards future directions in this interdisciplinary field. By focusing on current research trends, we can understand how innovations emerge and the implications of these developments on various applications.
Innovations in Microfluidic Designs
Recent innovations in microfluidic designs have revolutionized the way we conceptualize fluid dynamics. Designers are increasingly integrating complex functionalities within chips. This approach allows for enhanced performance in applications ranging from molecular diagnostics to drug delivery systems.
Some key elements of these innovations include:
- Multilevel networks: Researchers are now creating chips with multiple layers to enable simultaneous reactions and analyses. This increases throughput and efficiency.
- Smart materials: The incorporation of responsive materials into microfluidic devices is allowing systems to adapt their properties in real-time, significantly improving user experience.
- Modular designs: By developing modular designs, researchers enable easier customization of microfluidic systems to cater to specific applications.
These innovations are essential for expanding the capabilities of microfluidic devices and making them adaptable for various uses. The potential benefits are vast, fostering advancements in areas like personalized medicine and rapid diagnostics.
Advancements in Computational Methods
At the intersection of microfluidics and computation, significant advancements in computational methods play a pivotal role. Using computational algorithms to model fluid behaviors can significantly streamline design processes and optimize device performance.
Some advancements include:
- Machine Learning Models: Integration of machine learning algorithms allows researchers to predict fluid dynamics with greater accuracy, leading to optimized designs.
- Real-time data analysis: Current computational methods enhance the capability for real-time data collection and analysis. This is crucial for dynamic applications such as environmental monitoring and medical diagnostics.
- Simulation Tools: Advanced simulation platforms are enabling researchers to visualize and test microfluidic operations virtually. These tools reduce the time and costs traditionally incurred in experimental setups.
The advancement in these computational methods not only inspires new designs but also improves the overall reliability and efficiency of microfluidic systems. A synergistic approach between microfluidics and computational technologies is preparing a strong foundation for future applications.
"The intersection of microfluidics and computational methodologies shapes the next generation of diagnostic tools, bringing immense potential for efficiency and customization."
Future Prospects of Microfluidic Computing
The evolution of microfluidic computing holds great promise for transforming essential industries and research areas. Its ability to merge fluid dynamics with computational algorithms presents unique opportunities that could redefine data processing paradigms. This section will explore emerging applications and the integration of microfluidic projects with other technologies, shedding light on the manifold benefits and considerations that lie ahead.
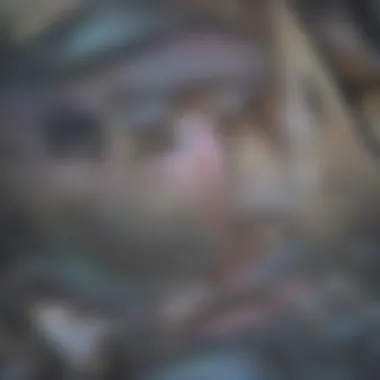
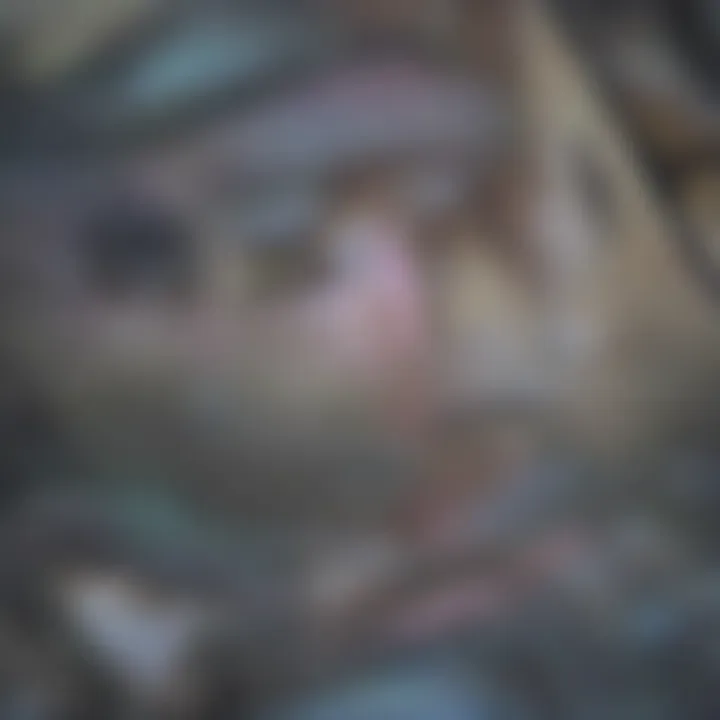
Emerging Applications
Microfluidic technology is poised to revolutionize various fields with new applications that were previously unattainable. One notable area is personalized medicine. With microfluidic devices, it is possible to conduct patient-specific drug tests, allowing for customized treatment plans that maximize efficacy while minimizing side effects. This is a significant step toward targeted therapies, taking into account the unique genetic makeup of individuals.
Another promising avenue is in environmental monitoring. Microfluidic systems can enable real-time detection of pollutants and pathogens in water supplies, enhancing public health responses. The small scale and efficiency of these devices make them ideal for on-site analysis, providing rapid feedback that traditional methods often lack.
Moreover, advancements in lab-on-a-chip technologies can enhance pharmaceutical research. By mimicking organ functionalities, these devices can expedite drug discovery and reduce the need for extensive animal testing. Researchers could create sophisticated models of human biological systems, yielding more reliable data that can accelerate the drug development pipeline.
"Microfluidics offers a powerful platform for creating innovative applications that address complex challenges across medicine and environmental science."
Integration with Other Technologies
The future of microfluidic computing will greatly benefit from its integration with other advanced technologies. For instance, combining microfluidics with artificial intelligence can enhance data interpretation and processing capabilities. AI algorithms can analyze the data produced by microfluidic devices, revealing patterns and insights that might be impossible to detect manually. This synergy could lead to breakthroughs in areas such as diagnostics and therapeutic monitoring.
Furthermore, the compatibility of microfluidic systems with biosensors and nano-devices opens new doors for health monitoring. Wearable devices equipped with microfluidic sensors could continuously assess vital biomarkers, delivering real-time health updates to both patients and healthcare providers. This development would not only empower patients but also improve preventative healthcare measures.
Incorporating microfluidics within the Internet of Things (IoT) ecosystem can also create interconnected systems for more comprehensive data collection and analysis. Smart sensors embedded in microfluidic devices could communicate data seamlessly, informing decisions in healthcare, agriculture, and environmental management. This interconnected approach enhances the overall efficiency and reliability of data-driven interventions.
As these technologies continue to converge, the potential for microfluidic computing becomes more expansive. Such progress will not only refine computational paradigms but also inspire innovative solutions to contemporary challenges in diverse fields.
Interdisciplinary Collaborations
Interdisciplinary collaborations are crucial to advancing the field of microfluidic computing. The integration of microfluidic devices into computational frameworks has opened new avenues that require the expertise from various disciplines. Science, technology, engineering, and mathematics (STEM) disciplines, along with social sciences and ethics, play pivotal roles in refining the applications and implications of microfluidic technologies.
Involvement of diverse fields such as biology, chemistry, and computer science in microfluidic computing enhances the understanding of biophysical processes while optimizing the design and functionality of microfluidic devices.
Partnerships Between Fields
Partnerships between different realms of study foster innovation in microfluidic computing. For instance, collaborations between biologists and engineers allow the creation of devices tailored for specific biomedical applications, yielding improvements in drug delivery systems and diagnostic tools. Such teamwork can lead to:
- Enhanced Problem Solving: Pooling expertise generates solutions that single-discipline teams might overlook.
- Broad Knowledge Exchange: Different perspectives enable the gathering of unique insights and innovation.
- Resource Sharing: Collaborations provide access to varied resources and instrumentation, essential for advanced research.
As researchers and practitioners from distinct backgrounds converge, they can address complex challenges faced in the development of microfluidic systems, honing the efficacy and reliability of the technology.
Influence on Research Paradigms
The influence of interdisciplinary collaborations on research paradigms is significant. By fostering a holistic view of microfluidics, the integration of methodologies from various fields propels the refinement and evolution of the technology. This cross-pollination leads to an era of research defined by:
- Innovative Approaches: New methodologies emerge from the collaboration of disciplines, enhancing experimentation and application.
- More Comprehensive Ethics Consideration: Engaging in interdisciplinary discourse can lead to more robust understanding of ethical implications necessary for responsible innovation.
- Policy Development: Engaging social scientists in the conversation allows for better understanding of the societal impacts and reinforces the importance of regulatory frameworks relevant to microfluidic technologies.
Such influences change not only how research is conducted but also its societal impact, assisting in redefining the standards and expectations within the microfluidics community.
Interdisciplinary efforts push the boundaries of knowledge, transcending traditional confines, and leading to profound advancements in microfluidic applications.
Ethical Considerations
As microfluidic technologies rapidly advance, ethical considerations become increasingly critical. The integration of computing with microfluidics presents new possibilities, but it also raises important questions regarding responsibility, privacy, and environmental impact. Understanding these aspects is vital for researchers, developers, and policymakers alike. In this discussion, we will focus on the implications of microfluidic technologies and the regulatory frameworks required to govern their application.
Implications of Microfluidic Technologies
Microfluidic technologies have profound implications not just for their immediate applications but also for society at large. These devices are capable of analyzing biological fluids, which can lead to advances in healthcare diagnostics and treatment options. However, with these advancements come several ethical considerations:
- Data Privacy: Personal health information derived from microfluidic devices must be handled with extreme care. Ensuring that patient data is anonymized and secure from unauthorized access is necessary to maintain trust in these technologies.
- Accessibility: There is a risk that advanced microfluidic technologies may not be accessible to all populations. This raises ethical issues around equity in healthcare, particularly in under-resourced areas.
- Environmental Impact: The materials used in developing microfluidic devices must be assessed for their environmental consequences. Sustainable practices in sourcing and disposing of these materials need to be established to minimize ecological harm.
The accountability of developers and manufacturers in addressing these issues is crucial. Avoiding exploitation while maximizing the benefits of these technologies must be a priority.
Regulatory Frameworks
With the evolving landscape of microfluidic technologies, establishing regulatory frameworks is imperative. These frameworks should aim to ensure safety, efficacy, and ethical compliance as these systems become more integrated into various industries. Considerations for regulatory approaches include:
- Standardization: Developing standards for microfluidic device design and manufacturing is necessary to ensure consistent performance and reliability. Regulatory bodies may need to set benchmarks that all devices must meet, ensuring safety for users.
- Evaluation Processes: Regulatory agencies must implement processes for evaluating the safety and effectiveness of microfluidic products. This includes rigorous testing and validation stages, similar to those used in pharmaceuticals and medical devices.
- Post-market Surveillance: After deployment, continuous monitoring of microfluidic devices is essential. This practice ensures they continue to meet safety standards and perform as intended over time.
- International Collaboration: As microfluidic technologies may cross borders, international cooperation in regulation can help standardize practices globally. This can prevent a patchwork of rules across different regions that can complicate development and distribution.
Establishing effective regulatory frameworks will ensure that the potential of microfluidic technologies is harnessed responsibly, promoting innovations while safeguarding public interests.
The End
The conclusion encapsulates the central ideas presented throughout this article on microfluidic computers. It emphasizes the necessity to understand how this technology integrates fluid dynamics with computational paradigms. This integration represents a significant evolution of traditional computing methods, offering advantages that are particularly relevant in various sectors including biomedicine and environmental monitoring.
Microfluidic computers operate with a remarkable level of precision and efficiency. They hold the potential to perform complex computations at a scale that is unachievable with conventional electronics. This has profound implications for medical diagnostics, where rapid and accurate data can lead to improved patient outcomes. In environmental monitoring, the ability to perform real-time assessments of water quality can aid in safeguarding public health and preserving ecosystems.
Moreover, as this technology continues to evolve, ethical considerations surrounding its applications will warrant careful attention. Stakeholders must be aware of the ramifications of deploying microfluidic systems in sensitive areas, such as healthcare or environmental protection. Regulatory frameworks will need to evolve in tandem with technological advancements to ensure safety and efficacy.
As we conclude, it is vital to recognize that the journey of microfluidic computers is still in its early stages. The ongoing research and innovation in this field promise a future where these systems can redefine data processing methods further.
Summary of Key Points
- Integration of Fluid Dynamics with Computing: Microfluidic computers represent a novel way of processing data through fluid dynamics, functionalizing systems on a microscale.
- Applications Across Sectors: The impact of this technology is evident in various fields, particularly in biomedicine for diagnostics and environmental science for monitoring.
- Ethical and Regulatory Developments: The advancements necessitate a deeper reflection on the ethical implications and the need for evolving regulations.
- Future Research Opportunities: Continuous innovation in microfluidic designs and computational methods will drive future developments.
Towards a Microfluidic Future
Looking ahead, the future of microfluidic computing appears promising. Emerging applications will likely explore areas not yet fully understood. For instance, advances in the integration of artificial intelligence with microfluidic systems could bring about more sophisticated data analysis capabilities.
We can anticipate a growing interest in hybrid systems that combine microfluidics with other technologies, such as nanotechnology or biotechnology. This convergence could enhance the performance and expand the applications of microfluidic computers, making them increasingly versatile.
Furthermore, interdisciplinary collaborations will play a critical role. Partnerships between engineers, scientists, and ethicists can lead to responsible innovation, ensuring that the benefits of microfluidic computing are maximized while addressing any potential drawbacks. As new frontiers in research and application emerge, the landscape of microfluidic computing will continue to evolve, potentially leading to groundbreaking discoveries and impactful solutions in the years to come.