In-Depth Analysis of Scintillators: Properties and Advances
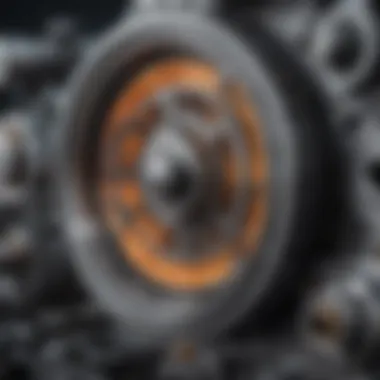
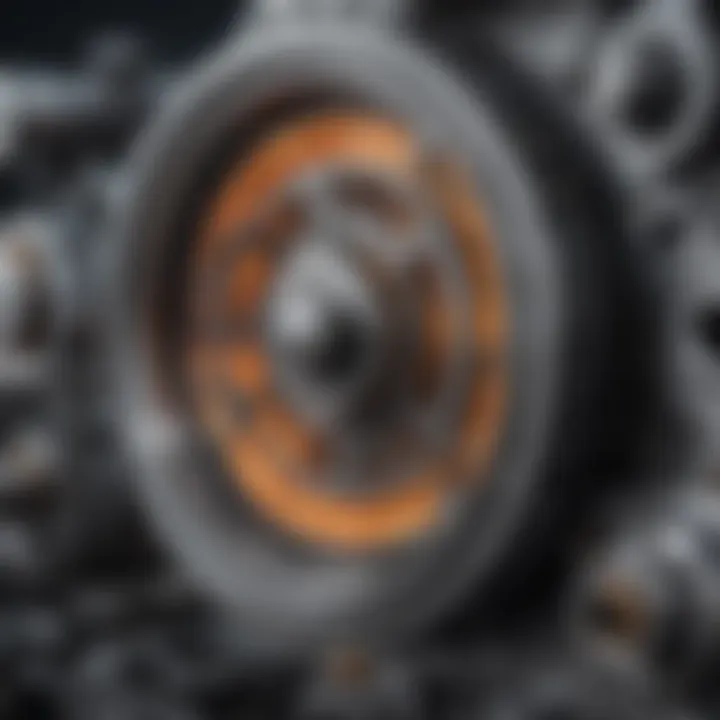
Intro
Scintillators are materials that have a unique ability to emit light when they interact with ionizing radiation. This interaction fascinates scientists and engineers alike, especially due to its wide-ranging applications. From nuclear physics to medical imaging, scintillators are integral in detecting various types of radiation. Understanding scintillator properties can lead to advancements in technology and practical usage in several fields.
In this article, we will explore the properties, applications, and recent advancements in scintillator technology. The aim is to provide readers with a thorough grasp of this complex subject, from the fundamental characteristics of scintillators to the cutting-edge innovations that are shaping the future of radiation detection.
Recent Advances
Recent developments in scintillator technology have shown promise in enhancing sensitivity and efficiency in radiation detection. This section will address both the latest discoveries and technological innovations in the field.
Latest Discoveries
New materials have been continuously studied for scintillation properties. Among them, the progress in organic scintillators has gained attention. These materials can offer benefits like light weight and cost-effectiveness compared to traditional inorganic scintillators such as sodium iodide or cesium iodide.
Recent research has also focused on improving the energy resolution of scintillators. For example, advancements in doped crystals aim to create better performance under high radiation levels, which is crucial for medical applications like PET scans. The combination of various compounds has proven successful in enhancing brightness and sensitivity.
Technological Innovations
Innovative technologies play a key role in the advancement of scintillators. For instance, advances in photodetector technology, such as silicon photomultipliers, have enabled more effective light collection from scintillation events. This improvement significantly enhances the detection of low-energy radiation.
Furthermore, novel manufacturing techniques, including 3D printing, are enabling the creation of scintillator shapes and sizes that were not feasible before. Such adaptations enhance their applicability in specific environments, such as portable radiation surveillance devices.
"The evolution of scintillator technology will expand the horizons in radiation detection, paving the way for future breakthroughs in security and healthcare sectors."
Methodology
To provide a detailed understanding of scintillators, a systematic approach is essential.
Research Design
This analysis is grounded in a review of existing literature, technological reports, and research studies. By synthesizing findings from various sources, the article aims to present a comprehensive overview of scintillator developments and their implications.
Data Collection Techniques
Data was gathered through multiple methods, including:
- Academic journals
- Technical reports from related industries
- Online resources such as Wikipedia, Britannica, and Reddit
This multi-faceted approach allows for a richer narrative, ensuring that the reader gains insight into both practical and theoretical aspects of scintillators.
By organizing the information systematically, the aim is to create an engaging and educational experience for anyone interested in the realm of scintillators, whether they are students, researchers, educators, or industry professionals.
Preamble to Scintillators
Scintillators play a crucial role in the detection of ionizing radiation. These materials emit light when struck by high-energy particles. Understanding scintillators aids in multiple disciplines, from basic research in nuclear physics to practical applications in medical imaging technologies. This section provides foundational knowledge on scintillators and sets the stage for deeper insights into their properties and applications. Recognizing how scintillators work is essential for advancing detection techniques and enhancing our comprehension of radiation-related phenomena.
Definition of Scintillators
Scintillators are materials that have the ability to convert ionizing radiation into detectable light. When radiation interacts with a scintillator, it excites the atoms within the material. This energy transition results in the emission of photons, usually in the visible spectrum. The emitted light can be measured by photodetectors, making scintillators vital in various scientific and industrial applications. These materials differ in their composition and efficiency, affecting their overall performance in detection systems.
History and Development
The history of scintillators dates back over a century. Early discoveries identified some organic crystals, like anthracene, as scintillating materials. Researchers first utilized these materials in the 1940s for radiation detection purposes. Over the years, advancements in technology and material science led to the development of many new scintillator types. Inorganic crystals, such as sodium iodide doped with thallium, emerged as prominent candidates due to their superior light output and energy resolution. The evolution of scintillator materials aligns with the growing needs of medical imaging and radiation detection, highlighting the ongoing significance of these substances in scientific research and application.
Physical Properties of Scintillators
Understanding the physical properties of scintillators is fundamental for grasping how these materials interact with ionizing radiation and convert that energy into detectable signals. These properties influence not only the efficiency of the scintillator but also its suitability for various applications. Key elements include light yield, decay time, and energy resolution, which determine the performance of scintillators in real-world scenarios.
Material Composition
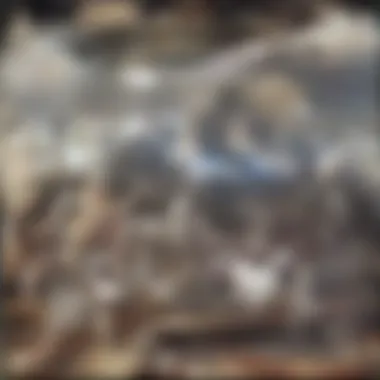
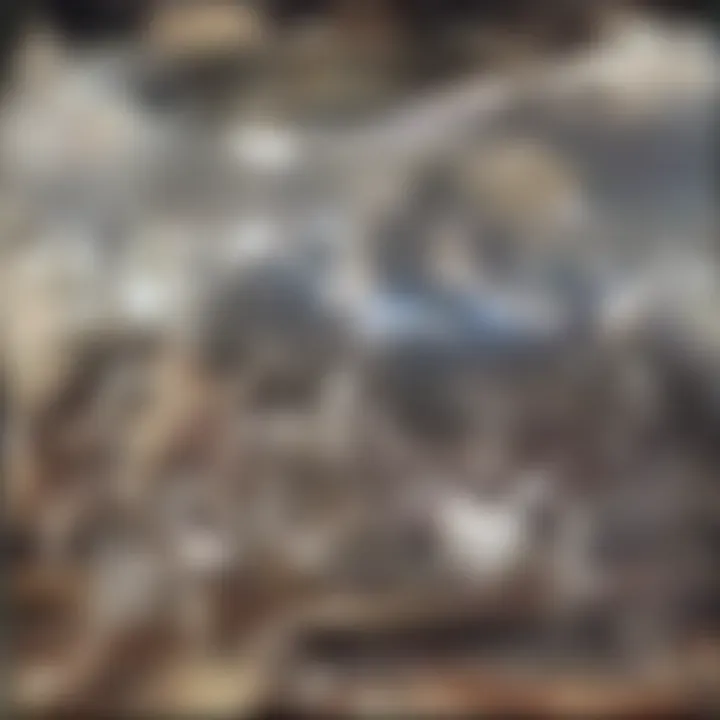
The material composition of scintillators is a crucial aspect as it directly impacts their effectiveness. Commonly, scintillators are made from two main categories: organic and inorganic materials.
- Organic Scintillators: These typically consist of hydrocarbon compounds. They are known for high light yield and shorter decay times. Examples include polystyrene and plastic scintillators that are lightweight and flexible, making them suitable for various applications.
- Inorganic Scintillators: These comprise crystalline compounds such as sodium iodide (NaI) and cesium iodide (CsI). They often have a higher atomic number, contributing to better photon interactions. Inorganic scintillators generally provide superior energy resolution compared to organic ones.
The choice of material plays a significant role in how well a scintillator performs in detection tasks. Factors such as cost, availability, and environmental stability also enter into consideration during material selection.
Chemical Characteristics
Chemical characteristics of scintillators can significantly affect their performance. This includes factors such as the stability under radiation exposure and the ability to reabsorb emitted light. High stability allows scintillators to function effectively over long periods, especially in demanding environments like those found in nuclear physics research.
Moreover, the optical clarity and transparency of the material determine how efficiently light can escape from the scintillator after an interaction occurs. The right combination of chemical properties ensures optimal signal output when radiation interacts with the scintillator.
In summary, understanding the physical properties, including material composition and chemical characteristics, is critical for advancing scintillator technology and optimizing their applications in detection and imaging.
"The performance of scintillators hinged on their material properties is indisputable, impacting both their detection capability and application range."
These factors are pivotal for researchers and professionals involved in the development and application of scintillator technology, underscoring the importance of well-informed material choices.
Mechanisms of Scintillation
Understanding the mechanisms of scintillation is crucial for grasping how scintillator materials operate. These mechanisms elucidate how incoming ionizing radiation gets converted into detectable signals, which is vital in various applications, such as medical imaging and nuclear physics research. The mechanisms primarily involve energy transfer processes and photon emission. Each of these elements plays a significant role in determining the efficiency and effectiveness of scintillator materials.
Energy Transfer Process
The energy transfer process is the initial step in scintillation. When ionizing radiation interacts with a scintillator material, it excites the atoms or molecules within. This interaction causes the material to absorb energy, leading to the formation of excited states. In simple terms, when radiation hits a scintillator, it gives energy to the material.
- Absorption of Energy: The ionizing radiation, such as gamma rays or alpha particles, transfers some of its energy to electrons in the scintillator. This energy transfer is essential because it initiates the scintillation process.
- Excitation: Once the electrons absorb this energy, they move from a lower energy level to a higher one. This stage is where the atoms become excited. The excited electrons remain in these states for a brief time before returning to their normal states.
- Thermalization: In certain scintillator materials, the excess energy may not translate directly into visible light. Instead, it may dissipate as heat, especially in materials with high atomic numbers. This factor can impact the efficiency of scintillator materials and is often a consideration in material selection.
Understanding the energy transfer process helps in selecting the right material for specific applications. More efficient energy transfer leads to better performance in detecting radiation.
Photon Emission
Once excitation occurs, the next step is photon emission, where the excited electrons return to their ground states. This process produces light, which is critical for detection.
- Decay of Excited States: The electrons emit photons as they transition from higher to lower energy levels. The wavelength of these photons can vary, influencing the type of scintillator used for specific applications.
- Luminescence: The light produced can be classified under various luminescence types such as fluorescence or phosphorescence. Fluorescence happens almost instantly, while phosphorescence involves a delayed emission, as energy is released over a longer period. This feature can be advantageous or disadvantageous depending on the application's requirements.
- Light Yield: The light yield of a scintillator material is essential for effectiveness. It refers to the amount of light produced per unit of absorbed energy. Higher light yields result in better detection capabilities, crucial for precise measurements in medical imaging systems like Positron Emission Tomography (PET).
"The efficiency of scintillation is significantly dependent on both the energy transfer process and the resultant photon emission, impacting how effectively radiation can be measured."
In summary, the mechanisms of scintillation encompass both the energy transfer and photon emission processes. Their understanding provides valuable insights into the behaviors and performance of scintillator materials. Such knowledge is essential for advancements in both technology development and practical applications in diverse fields.
Types of Scintillator Materials
Understanding the types of scintillator materials is crucial for several reasons. Each type offers specific properties and benefits that can significantly enhance the efficiency of detection systems. Moreover, different applications may require specific scintillator characteristics, thus making it important to choose the right material for the right use case. The classifications generally fall into three main categories: organic scintillators, inorganic scintillators, and hybrid scintillators.
The choice of scintillator material can impact aspects such as light yield, timing resolution, and energy resolution. Knowing the strengths and weaknesses of each type allows researchers and professionals to make informed decisions when designing experiments or applications.
Organic Scintillators
Organic scintillators are typically composed of carbon-based compounds, which include materials such as stilbene, anthracene, and polyvinyl toluene. One advantage of organic scintillators is their relatively high light yield, which can improve the efficiency of radiation detection. They can be readily produced in various shapes and sizes, making them adaptable for diverse applications.
However, organic scintillators also present challenges. They are often sensitive to temperature changes, which can affect their performance. Additionally, they can suffer from radiation damage over time, leading to degradation in their scintillation properties. Despite these limitations, organic scintillators find applications in various fields, including medical diagnostics and particle physics research.
Inorganic Scintillators
In contrast, inorganic scintillators are composed of crystalline compounds, such as sodium iodide doped with thallium (NaI(Tl)), cesium iodide (CsI), and lanthanum bromide (LaBr3). Inorganic scintillators usually exhibit superior energy resolution compared to organic ones, making them particularly suitable for applications requiring precise measurements such as spectroscopy.
The durability of inorganic scintillators can often be higher than that of organic scintillators. However, these materials can be more expensive and may have lower light yields. The density of inorganic materials also plays a role in shielding and radiation detection. Overall, inorganic scintillators are widely used in gamma cameras and radiation monitoring systems.
Hybrid Scintillators
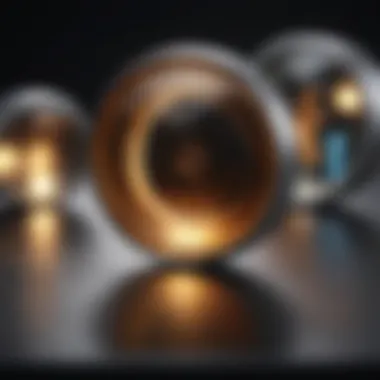
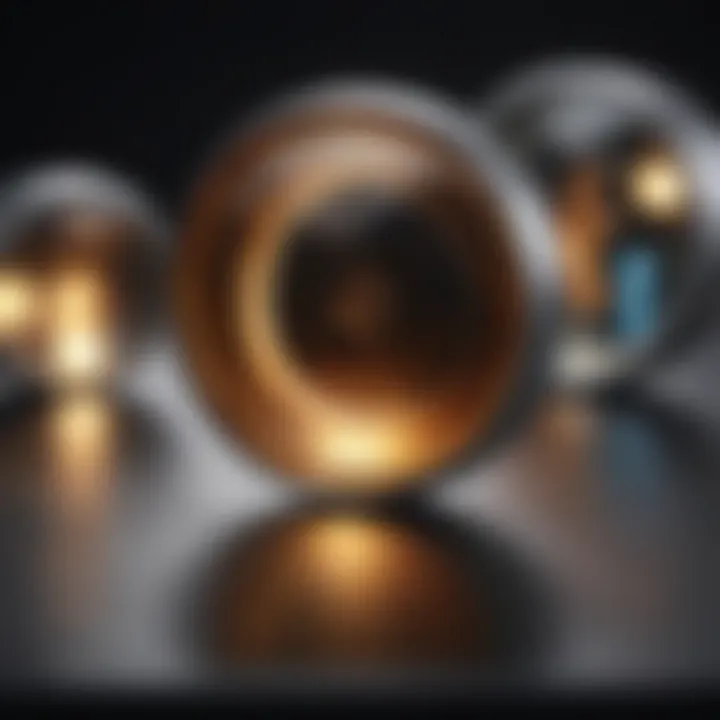
Hybrid scintillators represent a blend of both organic and inorganic materials, aiming to capture the advantages of each type. An example would be the use of organic materials for a faster response time combined with inorganic materials for improved energy resolution. These hybrid systems show promise in advancing detection technologies.
The potential of hybrid scintillators to be tailored for specific applications makes them an area of active research. Their capability for faster photon emission can enhance the overall performance in various detection systems. However, challenges remain regarding cost-effectiveness, which could hinder broader adoption.
The choice of scintillator material should not be taken lightly, as each type offers unique properties that can dramatically influence the outcome of research and applications.
Applications of Scintillators
Understanding the applications of scintillators provides crucial insight into their role in various technological and scientific fields. Scintillators transform the energy from ionizing radiation into observable light. This capacity makes them important in diverse sectors, including medicine, nuclear physics, and radiation protection. Introducing scintillators into these fields has considerably enhanced effectiveness in detecting radiation and analyzing energy levels. This section emphasizes notable applications, benefits, and some considerations regarding scintillator use.
Medical Imaging
Medical imaging is one of the most significant applications of scintillators. They are fundamental components of advanced diagnostic techniques. The ability to visualize internal body structures is essential in modern medicine. Scintillators facilitate the detection of radioactive tracers, aiding in the diagnosis of diseases and conditions.
Positron Emission Tomography (PET)
Positron Emission Tomography, commonly called PET, represents a standardized diagnostic tool that utilizes scintillators. It plays a pivotal role in oncology, cardiology, and neurology. The key characteristic of PET is its ability to provide 3D images of functional processes within the body. This functionality is particularly beneficial because it helps doctors spot cancer earlier than traditional imaging techniques.
A unique feature of PET scanning is its use of radiopharmaceuticals, which emit positrons. When these positrons meet electrons in the body, they annihilate each other, producing gamma rays that a scintillator detects. The advantages of this method lie in its precision and detailed images, which allow for more informed decisions in patient care. However, the primary disadvantage is the need for radioactive materials, which must be handled with care.
Single Photon Emission Computed Tomography (SPECT)
Single Photon Emission Computed Tomography, or SPECT, is another vital imaging technique that involves scintillators. This method is particularly noteworthy in cardiac imaging and evaluating brain disorders. Similar to PET, SPECT generates images of the distribution of radioactive substances in the body.
The key characteristic of SPECT lies in its use of gamma-ray-emitting radioisotopes. These isotopes are injected into the body, and scintillators capture the emitted gamma rays, forming images that reflect tissue and organ function. One unique feature of SPECT is its ability to provide information about blood flow and activity levels in various organs. The advantage is a relatively lower cost compared to PET. However, SPECT may not achieve the same resolution as PET.
Nuclear Physics Research
Scintillators are vital in nuclear physics research, where they detect high-energy particles and gamma rays. Researchers utilize scintillators in particle accelerators and detectors to analyze physical phenomena. The properties of scintillators differ depending on the materials used, affecting their performance in various applications. Researchers must consider aspects such as energy resolution, light yield, and decay time when selecting scintillator materials for specific experiments. Achieving rational knowledge in this field enables scientists to advance our understanding of fundamental physics and discover new particles.
Radiation Protection
Scintillators play an essential role in radiation protection. In various industries, including nuclear power and healthcare, maintaining safety from radiation exposure is crucial. Scintillator-based detectors are employed to monitor radiation levels in environments where radioactive materials are present. These detectors provide real-time measurements and enable prompt responses to potential hazards.
One significant aspect of using scintillators in this context is their ability to discriminate between different types of radiation. This characteristic helps safety professionals assess occupational and environmental exposure more accurately. In addition, scintillator-based systems allow for remote monitoring, which enhances safety practices.
The importance of scintillator applications is evident across several domains, from medical imaging advancements to essential roles in research and safety. Understanding their diverse uses enables professionals to harness their potential effectively.
Advancements in Scintillator Technology
Advancements in scintillator technology play a crucial role in expanding the capabilities and applications of scintillators. As the demands for high-performance detection systems increase, researchers are focusing on developing novel materials and innovative detection strategies. These advancements not only enhance the efficiency and sensitivity of scintillation-based methods but also open new avenues for research across various fields.
Emerging Materials
The continuous search for emerging materials is central to the evolution of scintillator technology. Traditional scintillators, such as sodium iodide and plastic scintillators, have served well historically, but limitations prompt the exploration of new alternatives. Recent developments have led to the introduction of materials such as cesium iodide doped with thallium and the use of organic-inorganic hybrid structures. These materials exhibit improved light yield, faster response times, and superior energy resolution, making them suitable for demanding applications.
- Metal Halide Crystals: These materials offer excellent scintillation properties. They are less sensitive to temperature changes compared to traditional scintillators, which is a significant advantage in variable environments.
- Nanostructured Scintillators: Advances in nanotechnology have enabled the fabrication of scintillators with enhanced properties at the nanoscale. These include higher light output and better radiation hardness, which are essential for applications in high-radiation environments.
- Polymer-based Scintillators: These materials combine flexibility and lightweight characteristics with effective radiation detection capabilities. Their ease of fabrication allows for innovative shapes and sizes tailored to specific needs.
Exploring these emerging materials opens up a broad spectrum of research opportunities. Their unique properties can contribute greatly to fields like medical imaging, radiation detection, and nuclear physics, improving existing technologies and paving the way for new applications.
Innovative Detection Strategies
Alongside material advancements, innovative detection strategies are transforming scintillator applications. The integration of advanced technology with scintillation detection leads to more accurate and efficient systems. Modern approaches leverage digital signal processing and machine learning to enhance data analysis from scintillation events.
- Time-of-Flight Measurement: This method utilizes the time difference in photon arrival to accurately determine the energy of incident radiation. Improved scintillator speed facilitates high temporal resolution in experiments.
- Multiphoton Detection Systems: By deploying arrays of detectors, these systems can capture multiple scintillation events simultaneously, greatly increasing throughput.
- Hybrid Detection Techniques: Combining scintillator detectors with complementary technologies such as CMOS sensors allows for real-time imaging and analysis. This fusion enhances the resolution and reduces noise in data collection, making it particularly valuable for medical imaging applications.
"The advancement in detection strategies not only improves the sensitivity and accuracy of scintillation systems but also allows for sophisticated analysis that was not possible before."
The innovations in detection strategies ensure that scintillator technology remains relevant in a fast-evolving landscape of scientific research and application demands. As the field continues to grow, it will increasingly rely on these advancements to push the boundaries of what is possible with scintillation-based technologies.
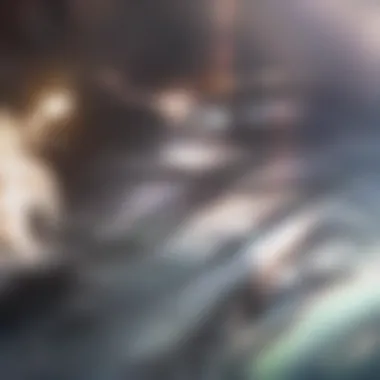
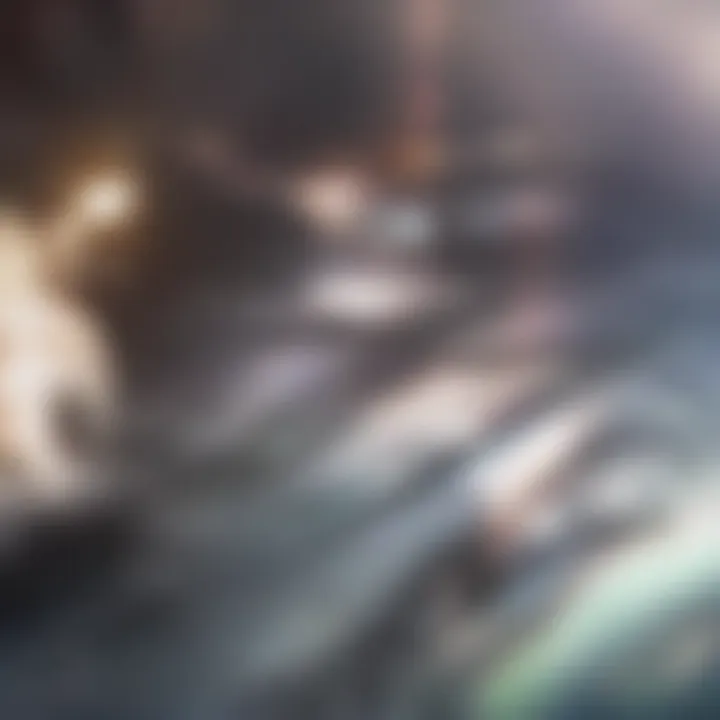
Limitations of Scintillator Materials
Understanding the limitations of scintillator materials is crucial for researchers and engineers. These limitations can significantly affect the performance and applicability of scintillators in various fields, including medical imaging and radiation detection. Being aware of these constraints can lead to improvements in design and material selection, thereby enhancing efficiency and accuracy in applications that rely on scintillation.
Response Time
Response time is one of the most significant limitations in scintillator technology. It refers to the time taken for a scintillator to emit light after being exposed to ionizing radiation. Fast response times are essential in scenarios like particle detection in high-energy physics. Organic scintillators generally exhibit quicker response times compared to their inorganic counterparts. However, this speed often comes at the cost of light yield and resolution.
In applications such as Positron Emission Tomography (PET), the detailed timings of emitted photons are critical for the accuracy of imaging. If the response time is too slow, the collected signals may overlap, leading to inaccuracies in the reconstructed images. This limitation emphasizes the need for next-generation scintillator materials that can provide rapid light emission without compromising other performance metrics. Such advancements can significantly expand the range of applications and increase reliability in critical measurements.
Temperature Sensitivity
Temperature sensitivity affects scintillator performance and reliability. Most scintillator materials show a variation in luminescence based on temperature. In high-temperature environments, the efficiency and energy transfer mechanisms may degrade, causing a shift in emission wavelengths and reduced light output. This is a notable concern in applications like nuclear physics, where precision is required under varying conditions.
Therefore, understanding how temperature influences scintillator behavior is vital. Developers must consider these factors when designing systems for specific environments. It might be necessary to implement cooling systems or select materials that maintain efficiency across a broader temperature range. The goal is to enhance stability and performance regardless of external conditions, ensuring that scintillators can reliably operate in diverse settings.
"Acknowledging the limitations helps pave the way for better innovations in scintillator technology, leading to improved applications in both academia and industry."
In summary, recognizing the limitations of scintillator materials, particularly response time and temperature sensitivity, is essential. These factors influence the development of more efficient materials and systems for various applications, setting the stage for future advances in the field of scintillation.
Future Directions in Scintillator Research
The exploration of future directions in scintillator research is vital given the rapid advancements in science and technology. This sector is experiencing notable transformations that promise to enhance the efficiency and applicability of scintillators. By focusing on materials innovation and their integration with other technologies, researchers are contributing to significant breakthroughs. Understanding these avenues can reveal new potential in fields such as medical imaging, particle physics, and radiation detection.
Next-Generation Materials Development
Next-generation materials for scintillation possess the capacity to surpass the limitations of traditional materials. Current materials, while effective, often present challenges like energy resolution and durability. Researchers are actively investigating polymers, quantum dots, and novel inorganic compounds. The introduction of these materials can lead to highly sensitive and faster response times, which is crucial for applications demanding precision.
Some material advancements include:
- Halide Perovskites: These have shown promise in achieving high scintillation efficiency and low production costs.
- Plastic Scintillators: Their flexibility and versatility make them attractive for varied applications, particularly in portable devices.
- Nanocomposite Scintillators: Combining various materials at the nanoscale can yield better performance metrics and custom properties tailored for specific tasks.
The goal is to develop scintillators that not only deliver improved performance but also ensure environmental sustainability through better recyclability.
Integration with Other Technologies
Integrating scintillators with modern technologies can amplify their effectiveness and broaden their applications. For instance, combining scintillators with advanced imaging systems enhances the resolution and precision of medical scans. Techniques like machine learning could also be applied to optimize the detection algorithms in radiation measurement devices.
Benefits of this integration include:
- Enhanced Detection Capabilities: Integrating artificial intelligence allows for improved pattern recognition in complex data sets.
- Robust Systems: Scintillators combined with real-time monitoring technologies can lead to safer environments in nuclear facilities.
- Cost Efficiency: By utilizing existing systems in conjunction with scintillators, organizations can reduce costs while upgrading their detection capabilities.
Closure on the Role of Scintillators
The role of scintillators is crucial in various fields of science and technology. They serve as essential tools for detecting and measuring ionizing radiation. Their ability to convert radiation into visible light opens pathways for advanced research and practical applications. In this article, we explored the multifaceted nature of scintillators. We highlighted their physical properties, mechanisms of scintillation, types of materials, and diverse applications, providing insights into their importance in nuclear physics, medical imaging, and radiation safety.
Scintillators are not just materials; they are key components that bridge the gap between radiation and data interpretation. Their utility in detecting and analyzing radiation enhances the understanding of complex phenomena in physics. For instance, in medical imaging, scintillators facilitate high-resolution imaging techniques, aiding in early disease detection and treatment planning. Their role in nuclear physics research allows scientists to accurately measure energy levels and understand fundamental interactions.
Furthermore, advancements in scintillator technology promise to improve detection efficiency. The development of next-generation materials will likely enhance scintillator response and broaden their applicability. Emerging materials like organic scintillators and hybrid formats show potential in offering more sensitive and faster response times, meeting the demands of modern applications. It is important to consider the advancements in integration with other technologies, amplifying scintillator performance across various fields.
Emphasizing the significance of scintillators leads to a better grasp of their influence on contemporary scientific disciplines. The continued study and innovation of these materials are essential for driving future developments in radiation detection and measurement technology.
"Scintillators reflect the ongoing evolution of detection technologies, embodying the essence of scientific progress."
Summary of Key Points
To summarize, key elements discussed in this article include:
- Definition and History: Scintillators are materials that emit light when ionizing radiation interacts with them. Their development has evolved significantly over the past century.
- Physical and Chemical Properties: They possess distinct material compositions and chemical characteristics that affect their scintillation behavior.
- Mechanisms of Scintillation: Understanding energy transfer and photon emission processes is vital to grasp how scintillators operate.
- Application Domains: Scintillators are widely used in medical imaging, nuclear research, and radiation protection, showcasing their versatility.
- Technological Advancements: Exploring emerging materials and innovative detection strategies reveals the potential for enhanced performance in the future.
- Limitations: Identifying challenges, such as response times and temperature sensitivity, provides critical avenues for improvement.
Implications for Future Research
The exploration of scintillators has significant implications for future research. Developing next-generation materials is paramount. Emphasis should be placed on synthesizing scintillators with improved light output and faster decay times. Also, understanding the interplay between scintillator characteristics and environmental factors is crucial. Research should focus on enhancing resilience to temperature fluctuations and radiation damage.
Moreover, integration of scintillators with other technologies may lead to innovative detection systems. Potential areas of investigation might include combining scintillators with advanced electronics, machine learning algorithms, and imaging systems. Such synergies can pave the way for real-time radiation monitoring, making significant strides in medical diagnostics and safety protocols.
In summary, the future of scintillator technology is bright, underpinned by evolving materials science and interdisciplinary collaboration. Ongoing research in this field holds the promise of revitalizing detection methods and expanding applications, thereby solidifying scintillators as indispensable tools in science and technology.