Understanding Gas Chromatograph Mass Spectrometry
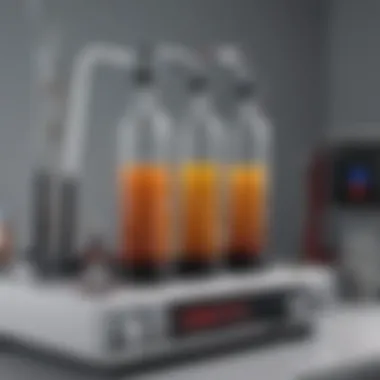
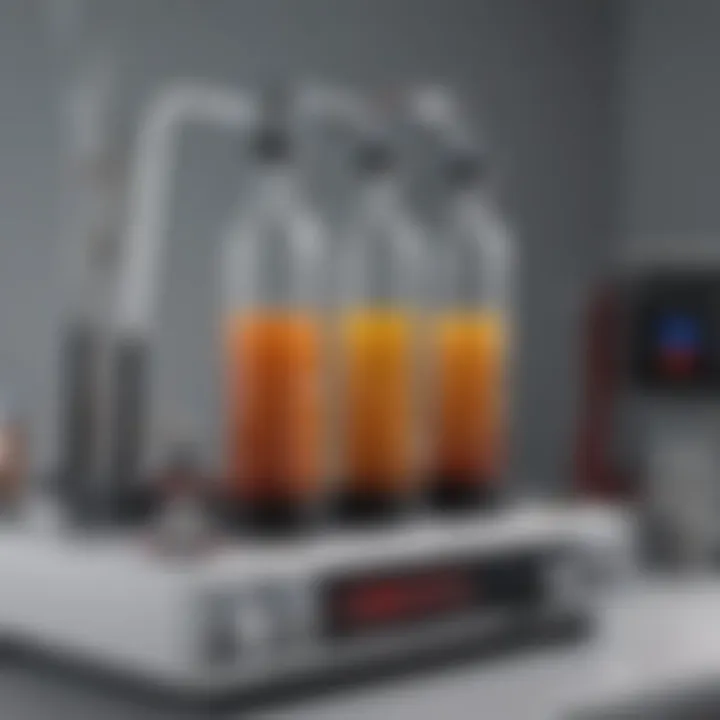
Intro
Gas chromatograph mass spectrometry (GC-MS) has become a cornerstone of analytical chemistry, functioning as a powerful tool in breaking down and analyzing complex mixtures. This method not only separates components of a sample but also provides information about their molecular structures through mass spectrometry. Its importance spans across various fields, such as environmental science, pharmaceuticals, and food safety, among others. This article aims to reveal the mechanics, applications, and challenges of GC-MS, offering insights critical for students, researchers, educators, and experts.
Understanding this technique involves grappling with its intricacies and appreciating its vast capabilities. The interplay of gas chromatography and mass spectrometry creates a diagnostic procedure capable of resolving some of the toughest analytical challenges.
Recent Advances
Latest Discoveries
Recent research has spotlighted the role of GC-MS in various unexpected niches. For instance, a study has indicated that this technique is increasingly being used to detect human metabolites in environmental samples. This has potential implications for public health, especially in assessing the exposure to pollutants through human activity.
Moreover, novel applications are emerging in the field of forensics. The ability to analyze trace residues from crime scenes can significantly enhance criminal investigations. By creating libraries of specific compounds, forensic scientists can tap into sophisticated comparison methods to ascertain connections between suspects and crime sites.
Technological Innovations
The technological strides seen in GC-MS are nothing short of impressive. One notable advancement is the development of miniaturized mass spectrometers, which retain sensitivity while offering portability. These devices enable fieldwork in remote locations, bringing analysis directly to the sample source. Furthermore, improvements in software algorithms have enhanced data analysis speed, fostering quicker decision-making in laboratories.
Such innovations facilitate the application of GC-MS in real-time monitoring setups, making it indispensable in industries where safety and compliance are paramount.
Methodology
Research Design
To understand the application of GC-MS effectively, itβs crucial to acknowledge the structured approach often adopted in research settings. Typically, studies begin with objective formulation, identifying the compounds of interest.
This is followed by developing a clear research design that incorporates the specific sample matrices. Whether soil, water, or biological tissues, the design must thoughtfully consider extraction methods and analysis protocols.
Data Collection Techniques
The data collection process in GC-MS is intricate. It begins with sample preparation, which may involve extraction and concentration methods, ultimately leading to the injection of the sample into the gas chromatograph.
Key steps include:
- Quenching: Reducing or halting chemical reactions.
- Derivatization: Modifying the chemical composition for better detection.
- Calibration: Establishing reliable response factors for quantitative analysis.
Each technique plays a vital role in ensuring that the analysis yields accurate and reproducible results, crucial for validating findings in both research and applied settings.
"The precision of GC-MS in quantitative analysis is often unparalleled, making it a go-to analytical method in various sectors."
Foreword to Gas Chromatograph Mass Spectrometry
Gas Chromatograph Mass Spectrometry, commonly known as GC-MS, stands at the intersection of gas chromatography and mass spectrometry, forming a robust analytical technique that is invaluable in various fields like environmental science, pharmaceuticals, and clinical diagnostics. This technique allows scientists and researchers to separate, identify, and quantify compounds in complex mixtures, making it a staple in analytical chemistry. The precision and resolution offered by GC-MS enable the detection of trace amounts of substances, thereby enhancing our ability to analyze diverse samples effectively.
In this article, we aim to delve into the fundamental mechanisms behind GC-MS, its components, and its extensive applications. By unpacking these elements, we can better appreciate why GC-MS is touted as one of the leading techniques in analytical chemistry.
Historical Context
The pivotal moments that led to the birth of GC-MS date back to the mid-20th century. The initial concepts of gas chromatography were pioneered by scientists like Archer John Porter Martin and Richard Laurence Millington Synge, who were awarded the Nobel Prize in Chemistry in 1952. Their inventive spirit paved the way for further advancements, ultimately leading to the integration of mass spectrometry with gas chromatography in the 1960s. This melding of technologies offered a new avenue for analyzing volatile compounds with unmatched accuracy.
GC-MS became increasingly prominent over the years due to its adaptability and precision in separating and identifying complex mixtures. Ever since its inception, researchers have continuously evolved and enhanced this technique, leading to innovations in instrumentation, ionization methods, and data interpretation strategies.
Defining GC-MS
At its core, Gas Chromatograph Mass Spectrometry is a hybrid method that utilizes the strengths of both gas chromatography and mass spectrometry. Essentially, gas chromatography separates the compounds in a sample based on their volatility, while mass spectrometry identifies and quantifies them based on their mass-to-charge ratio.
The process begins with a sample being vaporized and injected into the gas chromatograph, where it is carried through a column by an inert gas. The different components of the mixture move through the column at varying rates, allowing for their separation. Once the compounds elute from the column, they enter the mass spectrometer, where they are ionized and fragmented into charged particles. These ions are then analyzed, revealing valuable information about the chemical composition of the original sample.
In summary, the integration of gas chromatography and mass spectrometry not only facilitates the in-depth analysis of complex mixtures but also allows researchers to tackle a broad spectrum of analytical challenges across various disciplines. This holistic approach makes GC-MS a vital tool in modern scientific inquiry.
Fundamentals of Gas Chromatography
Gas chromatography stands as a cornerstone in analytical chemistry, providing a robust method for separating and analyzing compounds within a mixture. The importance of this topic lies not only in its foundational concepts but also in its integral role in the subsequent integration with mass spectrometry to form the powerful GC-MS technique. Understanding the fundamentals of gas chromatography allows one to appreciate how substances are identified and quantified in various fields, from environmental monitoring to pharmaceuticals.
Several key elements are crucial in grasping the principles of gas chromatography, from its operational mechanics to its benefits and the considerations one must take into account. The technique operates on the basic premise of separating volatile compounds based on their partitioning between a stationary phase and a mobile phaseβin this case, a gas.
Benefits of gas chromatography include:
- High resolution: This enables the distinction between closely related substances.
- Speed: Rapid separations can be achieved, making it ideal for high-throughput settings.
- Versatility: Applicable to various sample types and industries, enhancing its relevance in research and everyday applications.
Clearly, the fundamentals provide a lens through which the sophistication of GC-MS can be appreciated while highlighting the considerations essential for effective technique application.
Principles of Gas Chromatography
At the heart of gas chromatography are its foundational principles, which dictate how separation occurs. The principle can be distilled down to the interaction between different compounds and the phases involved. Usually, a sample is vaporized and carried through a column by an inert gasβa mobile phase. The separation occurs primarily due to differences in each compound's volatility and interaction with the stationary phase lining the column.
This interaction leads to varying travel times for different components, with some compounds eluting faster than others, allowing for a clean separation.
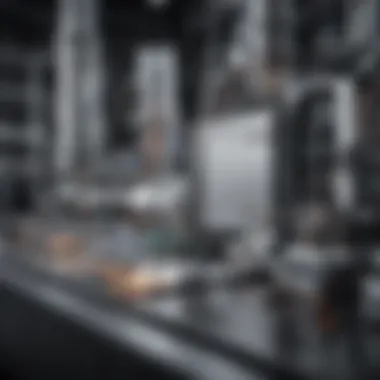
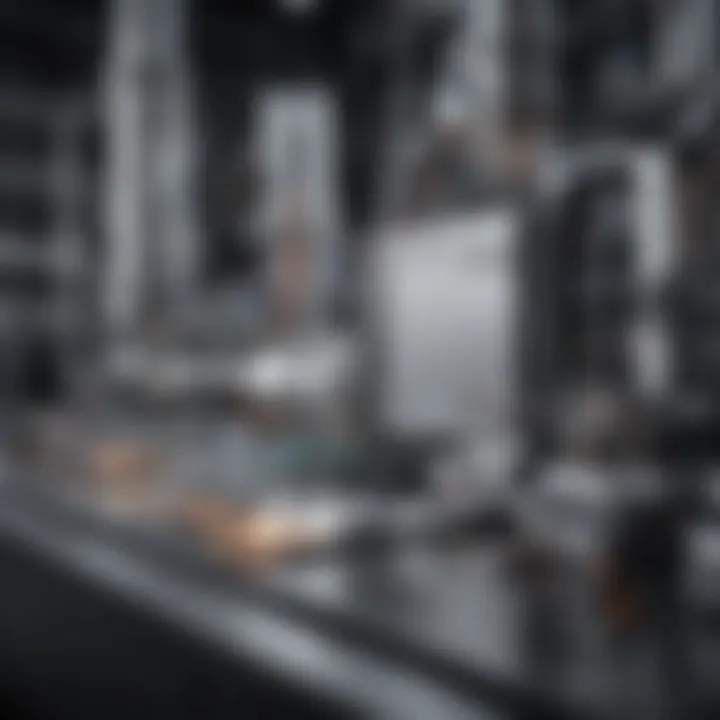
Components of a Gas Chromatograph
Understanding the components of a gas chromatograph is essential for anyone looking to master this technique. Three main components make up the system: the injector, the column, and the detector. Each has specific roles and characteristics that contribute to the overall effectiveness of the gas chromatograph.
Injector
The purpose of the injector is crucialβit introduces the sample into the chromatography system. Often seen as the gateway for samples, the injector must vaporize the substances efficiently. One key characteristic of injectors is their ability to handle small sample volumes while maintaining sample integrity.
For instance, a common type of injector is a split/splitless injector. This design allows for flexibility in how much of a sample is introduced into the column, accommodating various sample concentrations.
The versatility of injectors plays a significant role in ensuring that the gas chromatograph can adjust to the specific requirements of the analysis being carried out.
However, there's a unique feature to note: injectors also influence the temperature settings crucial for vaporization. If not set correctly, this might lead to loss of volatile compounds or inadequate vaporization. This can ultimately affect the quality of analytical results, making careful calibration paramount.
Column
The column is where the magic happens in gas chromatographyβit's where the actual separation of compounds takes place. A significant aspect of the column is the stationary phase material, which can vary from polar to non-polar types. This flexibility is what makes the column a beneficial choice in different applications.
A popular choice among researchers is the capillary column because of its high resolution and efficiency. Its advantages simply cannot be overlookedβthe narrow diameter means better separation efficiency and lesser sample consumption. But there's a flip side: they can be more challenging to handle and may require specialized equipment.
Detector
Lastly, we arrive at the detector, a pivotal component in confirming the identity and quantity of the separated components. The most common type of detector used in GC is the Flame Ionization Detector (FID), which is renowned for its sensitivity to hydrocarbons. One key characteristic of the FID is its ability to detect compounds at very low concentrations, making it especially beneficial for trace analysis.
Unique features of detectors may include response times and sensitivity. While FIDs are excellent for certain compounds, they may not effectively respond to non-combustible gases. Thus, the choice of detector depends heavily on the chemicals likely to be analyzed.
Separation Mechanisms
Separation in gas chromatography relies on a phenomenon known as adsorption, where molecules adhere to the stationary phase within the column based on their unique chemical properties. Two primary mechanisms drive this process: partition and adsorption chromatography.
- Partition chromatography involves compounds distributing between two phasesβliquid and gas, based on solubility. Itβs quintessential for polar compounds, which have interactions with the liquid stationary phase.
- Adsorption chromatography, on the other hand, is where solid phases interact with analytes. It can separate non-polar components quite effectively, an important consideration when dealing with complex samples.
Mass Spectrometry: An Overview
Mass spectrometry plays a pivotal role in the field of analytical chemistry, serving as a fundamental technique for identifying and quantifying chemical compounds. Its importance in the context of gas chromatograph mass spectrometry (GC-MS) cannot be overstated. Since it functions as the second half of the GC-MS setup, mass spectrometry is vital for elucidating the composition of mixtures that gas chromatography separates. Understanding how mass spectrometry operates sets the stage for fully grasping the overall functionality of GC-MS.
This overview highlights both the contributions and benefits of mass spectrometry to the analytical discipline. Without this technique, many of the more intricate analyses performed in various applications, such as environmental monitoring, pharmaceutical development, and clinical diagnostics, would be virtually unattainable.
Conceptual Framework
At its core, mass spectrometry is based on the principle of breaking down molecules into smaller ions, which are then analyzed based on their mass-to-charge ratios. The fundamental concept here is to convert a compound into ions and separate these ions according to their mass. This capability allows for not just qualitative analysisβdetermining what a compound isβbut also quantitative assessmentβdetermining how much of that compound is present.
The framework of mass spectrometry encompasses several techniques that work together to achieve the desired information about the sample. This integrative nature of mass spectrometry is another key aspect that underscores its significance within GC-MS. In short, it provides a powerful tool for characterizing complex biochemical entities.
Mass Spectrometer Components
The mass spectrometer itself consists of several key components that each contribute to the overall process of mass spectrometry. Understanding these elements gives clarity to how mass spectrometry functions within GC-MS.
Ion Source
The ion source is where the conversion of neutral compounds into charged particles begins. It is fundamental to the mass spectrometry process. By using various ionization methods, this component allows for the flexibility needed to analyze a wide array of compoundsβfrom volatile to non-volatile substances.
A notable characteristic of ion sources is their ability to be tailored to specific requirements. For instance, Electron Impact Ionization tends to produce a lot of fragmentation, which can be useful for identifying smaller molecules but could lead to loss of some information for larger or more complex compounds. This gives the ion source significant weight in GC-MS results, impacting both sensitivity and specificity. The adaptability here makes this component a popular choice within the GC-MS framework.
Mass Analyzer
The mass analyzer serves the crucial job of measuring the mass-to-charge ratios of ions created in the ion source. Various designs, such as time-of-flight (TOF) or quadrupole systems, each come with their advantages and disadvantages.
One key feature of mass analyzers is their resolutionβ the ability to distinguish between two ions of similar mass. High-resolution mass analyzers can provide more detailed insights, which is particularly helpful when dealing with complex mixtures. If a study requires precise mass determination or the analysis of closely-related compounds, the mass analyzer becomes a focal point of consideration within the research design.
Detector
The detector is the final component that captures the ions post-separation. Its ability to convert the ion signal into a readable electrical signal determines the effectiveness of data output from the mass spectrometer. Different types of detectors, like the electron multiplier, excel at specific analyses, especially when it comes to low-abundance compounds.
A critical aspect of the detector is its sensitivity, which can significantly impact the limits of detection. This highlights its importance in studies requiring detection of minute amounts of substances, which is often the case in environmental or clinical diagnostics. The choice of detector can have cascading effects on the overall results garnered from the GC-MS process.
Ionization Techniques
Ionization techniques are another defining element of mass spectrometry, and their selection greatly impacts the quality of the analytical results.
Electron Impact
Electron Impact ionization is a widely-used technique that involves bombarding a sample with high-energy electrons. This method is particularly favorable for small and volatile organic compounds. Electron Impactβs ability to induce fragmentation can be helpful for structural elucidation, providing a wealth of mass spectral information. However, its major downside is that it may not effectively ionize larger, polar molecules.
The result is a rich profile that is beneficial for organic chemists but might not suit all applications. It exemplifies a balance of extensive molecular information with certain limitations that researchers must always be aware of when choosing their methodologies.
Electrospray Ionization
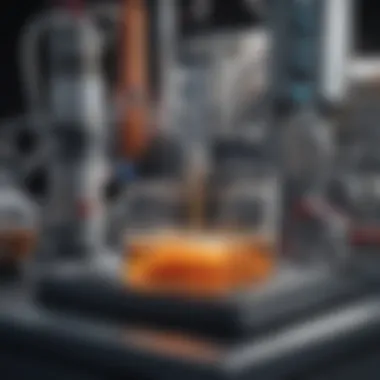
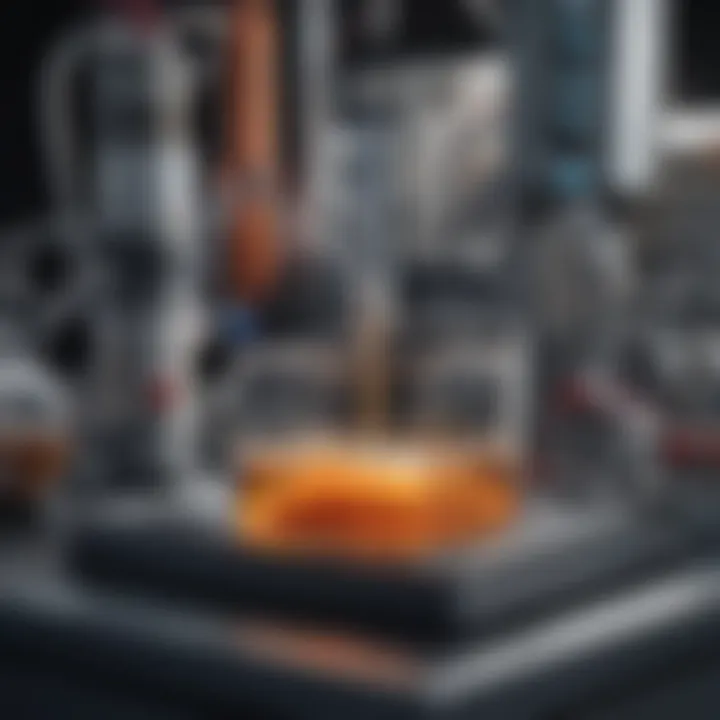
On the other end of the spectrum sits Electrospray Ionization (ESI), which allows for the analysis of larger biomolecules, including proteins and nucleic acids. It works by applying a high voltage to a liquid to create an aerosol of charged droplets. As these droplets evaporate, gas-phase ions are produced, which can then be analyzed.
A standout characteristic of ESI is its soft ionization process; it maintains the integrity of the original molecule without causing significant fragmentation. However, its main limitation lies in its dependency on solvent compatibility, as certain solvents can interfere with the ionization process. This balance makes ESI a popular choice for more high-molecular-weight analyses, particularly in fields like proteomics.
Above all, understanding the intricacies of these components and techniques paves the way for adopting mass spectrometry in various applications within todayβs scientific landscape. The depth of knowledge concerning the fundamental aspects of mass spectrometry ultimately enhances the robustness of the GC-MS methodology.
Integration of GC and MS
The integration of gas chromatography (GC) and mass spectrometry (MS) marks a significant advancement in analytical methodologies, enabling the precise identification and quantification of complex mixtures in a variety of samples. This combination is more than just a pairing of two methods; it creates a powerful analytical tool that broadens the horizons for researchers, educators, and industry practitioners alike.
How GC-MS Works
At its core, GC-MS operates in two distinct phases. First, the gas chromatograph separates the components of a sample based on their volatility. In this initial phase, samples are vaporized and injected into a column where they are separated based on their interaction with the column's stationary phase. Once separation occurs, the individual components exit the column and enter the mass spectrometer.
The mass spectrometer takes over from here. It ionizes the separated compounds, often through methods such as electron impact or electrospray ionization, allowing for the assessment of their mass-to-charge ratio. The resulting spectra provide detailed information about the chemical structure and concentration of the components, leading to a better understanding of the sample's composition.
Advantages of Combining Techniques
Enhanced Sensitivity
Enhanced sensitivity is a hallmark of the GC-MS combination, allowing detection of minute quantities of substances. With traditional GC methods, certain compounds may elude detection due to low concentration. However, the mass spectrometer compensates for this limitation. It can reliably detect compounds in the low-picogram level, making it a go-to choice for environmental monitoring or trace analysis.
This heightened sensitivity not only aids in detection but also contributes to the confidence scientists have in their data. With modern detector technology, such as the ion trap or quadrupole, even complex samples can be analyzed with pinpoint accuracy, delivering results that are often shockingly clear.
Improved Resolution
Improved resolution is another key benefit of combining GC and MS. This technical precision allows for more effective differentiation between compounds that may appear similar. In practice, improved resolution means that two closely eluting compounds can be identified as separate entities, which is vital in fields like pharmaceuticals and forensics.
A unique feature of this combination is that it leverages the strengths of each individual technique. Gas chromatography excels at separating complex mixtures, while mass spectrometry provides the ability to dissect the components at an elemental level. With enhanced resolution, researchers receive clear spectral data that clarifies complex plots, leading to more accurate conclusions in experimental results.
"The marriage of GC and MS has revolutionized analytical procedures, enabling scientists to identify substances with unprecedented clarity and sensitivity."
In summary, the integration of GC and MS creates a platform that not only enhances sensitivity and resolution but also informs future methodologies and applications. As analytical challenges continue to evolve, this powerful tool becomes increasingly relevant, making it a staple in laboratories around the world.
Applications of GC-MS
Gas chromatography-mass spectrometry (GC-MS) stands as a cornerstone in analytical chemistry. Its adaptability and precision make it invaluable across a variety of sectors. In this section, we will explore the specific applications of GC-MS, emphasizing how this technique not only enhances analytical capabilities but also contributes significantly to scientific progress.
Environmental Analysis
In environmental study, GC-MS is a game changer. It aids in the detection and quantification of pollutants, enabling scientists to monitor both air and water quality effectively. For instance, by identifying volatile organic compounds (VOCs) in air samples, researchers can assess pollution sources.
Moreover, GC-MS can analyze soil samples for pesticide residues, which is critical for agricultural sustainability and food safety. This dual capability of assessing both hazardous substances and their degradation products creates a fuller picture of environmental health.
- Key Points in Environmental Analysis:
- Detection of trace contaminants at low concentrations
- Identification of unknown compounds due to detailed spectra
- Regulatory compliance for pollutants, ensuring community health
In essence, the ability of GC-MS to offer fast and accurate results in environmental assessments not only helps in compliance with legal standards but also promotes proactive environmental management.
Pharmaceutical Research
The pharmaceutical industry widely employs GC-MS for drug development and quality control. During the development phase, researchers utilize GC-MS to determine the purity of compounds and to identify impurities. This is crucial as impurities can alter the safety and efficacy of medications.
The role of GC-MS extends to therapeutic drug monitoring. In clinical settings, it assists in measuring drug levels within patient samples, which informs dosage adjustments.
- Benefits of GC-MS in Pharmaceuticals:
- Comprehensive analysis of complex mixtures in formulation
- Fast turnaround times for regulatory submissions
- Support in bioavailability studies of new drugs
This detailed insight into pharmaceutical compounds underscores the unwavering commitment to safety and efficacy in medications, ensuring that medical practitioners can trust the prescribed treatments.
Clinical Diagnostics
In clinical diagnostics, GC-MS holds a significant role; it is particularly effective in the field of biomarker discovery. By analyzing metabolic profiles, GC-MS can detect diseases much earlier than some traditional methods. For example, it is instrumental in screening for metabolic disorders by identifying particular metabolites in urine or blood.
Furthermore, this technology enables accurate monitoring of conditions like diabetes or liver disorders through the analysis of metabolites associated with these diseases. It brings clarity where ambiguity often exists.
- Challenges and Considerations in Clinical Diagnostics:
- Requires a meticulous approach to sample preparation to avoid contamination
- Sophisticated interpretation of complex data sets needed for accurate diagnosis
- Cost implications in a clinical setting must be considered
Overall, the integration of GC-MS in clinical diagnostics represents a leap toward personalized medicine, providing healthcare practitioners with the tools to tailor their approach to individual patient needs.
Challenges in GC-MS Utilization
Gas Chromatograph Mass Spectrometry (GC-MS) is a powerful analytical tool widely recognized for its strengths in various fields. However, like any intricate system, it comes with its own set of challenges. Understanding these hurdles is essential for researchers and practitioners who wish to leverage the full potential of GC-MS. Addressing these challenges not only enhances the reliability and accuracy of data but also improves overall productivity in research.
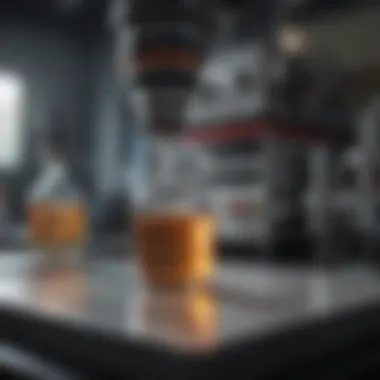
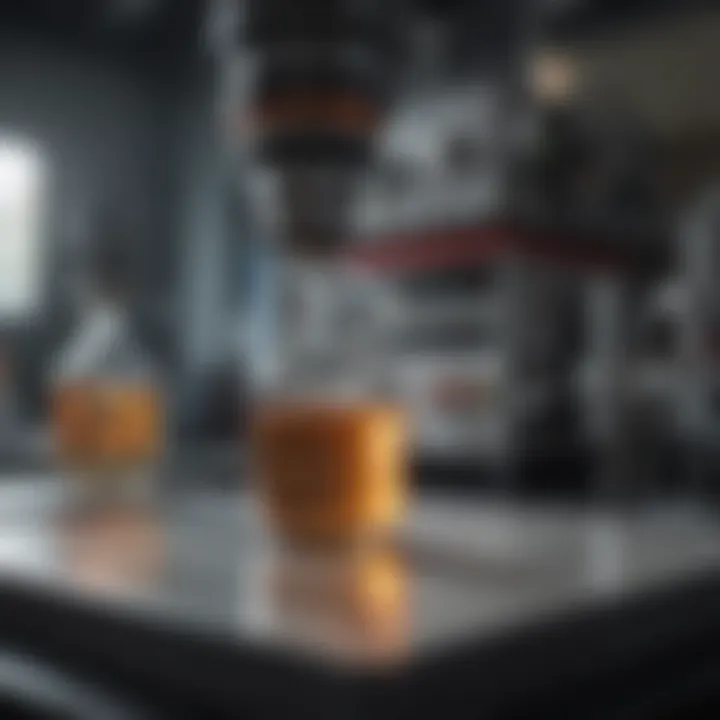
Instrument Calibration and Maintenance
Maintaining the accuracy of GC-MS instruments starts with proper calibration. Calibration ensures that the instrument produces valid and reproducible results. A failure in calibration can lead to significant errors, skewing the data and affecting the conclusions drawn from experiments. Regular maintenance routines should include checking the injector temperature, verifying mass spectrometer settings, and ensuring that columns are in optimal condition. Moreover, each of these components often has a specific calibration requirement based on its use and the type of samples being analyzed. Regular checks can prevent costly downtime and ensure smoother operations.
Data Interpretation Issues
Data interpretation in GC-MS is often a complex process, dictated by the intricate nature of the spectra generated.
Complex Spectra
Complex spectra are not just an occasional headache; they can be a daily challenge for many professionals using GC-MS. The multitude of overlapping peaks can make it tough to pinpoint specific compounds. This disorder arises, in part, from the complexity of the sample matrix and the varying ionization efficiencies of different substances. As a result, distinguishing between compounds can feel like finding a needle in a haystack.
The key characteristic of complex spectra is their inherent difficulty in distinguishing between various analytes. This complexity poses both a challenge and an opportunity. For instance, highly sophisticated software has been developed to facilitate more accurate peak identification and quantification. However, it requires a level of expertise to use effectively, often training individuals can be time-consuming and lead to a steep learning curve.
Quantitation Challenges
Quantitation challenges are another significant aspect that many encounter while working with GC-MS. Accurate quantification of substances in a sample is vital for research purposes, especially in the fields of environmental analysis and pharmacokinetics. The main difficulty lies in the need for precise calibration curves to ensure that the results reflect the true concentrations of compounds present.
Additionally, matrix effects can severely impact the quantitation process. Often, the presence of other substances can enhance or suppress the signals of the target analytes, leading to inaccuracies in the quantitated values. This makes developing robust and reliable methods even more time-consuming. In reality, balancing effectiveness and efficiency while ensuring accuracy is a constant battle for professionals working with GC-MS.
Sample Preparation Difficulties
Sample preparation remains a daunting task, underpinning the success or failure of any GC-MS analysis. Preparing a sample typically involves several steps, including extraction, concentration, filtration, and often, derivatization. Each of these stages can introduce variability, potentially influencing the quality of the data obtained.
For instance, improper sample extraction can leave behind unwanted compounds or dilute target analytes, while inefficient filtration can introduce particulates that interfere with the chromatographic process. Additionally, the time needed for thorough sample preparation stretches available resources, leading researchers to sometimes rush, which could compromise the data's integrity. Achieving a standardized protocol that is both effective and efficient is vital for improving the reliability of GC-MS methods.
Keeping an eye on the potential pitfalls of GC-MS utilization is crucial for anyone dealing with this powerful technique. The challenges associated with calibration, data interpretation, and sample preparation can shape the overall outcome of analytical results, emphasizing the importance of stringent protocols and ongoing training.
Future Perspectives on GC-MS
The world of analytical chemistry is ever-evolving, and gas chromatograph mass spectrometry (GC-MS) stands at the forefront of this evolution. As society faces complex challenges, the importance of GC-MS cannot be overstated. This section delves into future perspectives, focusing on technological advancements and the expansion of applications. It emphasizes how these elements shape the future of GC-MS and its significance in various fields.
Technological Advancements
Miniaturization
Miniaturization in the context of GC-MS is a game changer. Essentially, it involves developing smaller, more compact instruments without sacrificing accuracy or efficiency. This is particularly beneficial for fieldwork, where portability can be a real boon. The key characteristic of miniaturization is the ability to conduct analyses on-site, thus speeding up the decision-making process.
One unique feature of miniaturized GC-MS systems is their lower power consumption. This makes them not only more sustainable but also easier to operate in remote locations where energy resources are limited. The advantages here are manifold; quicker results, reduced costs, and increased accessibility for smaller labs and educational institutions, fostering broader research opportunities. However, itβs worth noting that designing these compact systems raises its own set of challenges, particularly concerning maintaining the sensitivity and resolution that traditional units offer.
Integration with Other Techniques
Integration with other techniques promises to broaden the horizons for GC-MS. Here, we are looking at leveraging complementary methods like liquid chromatography or tandem mass spectrometry. The significant characteristic of this integration is that it creates a platform for a more comprehensive analysis, enriching data quality and enhancing interpretability.
A noteworthy facet is that the combination enables the detection of a wider range of compounds, which can be especially useful in complex matrices. The inclusion of additional techniques can bolster sensitivity and selectivity, allowing scientists to obtain a fuller picture of their samples. However, the challenge lies in the increased complexity of data interpretation and the need for skilled personnel. Balancing these factors is essential as the analytical landscape continues to evolve.
Expanding Applications
Metabolomics
Metabolomics is a rapidly growing field that examines the unique chemical fingerprints left behind by cellular processes. Its synergy with GC-MS opens up fresh avenues for research, especially in understanding biological systems. The key characteristic of studying metabolites through GC-MS is the high sensitivity and specificity, allowing scientists to detect minute quantities that could be clinically relevant.
This combination holds great promise, particularly in clinical diagnostics and drug discovery. It provides insight into metabolic pathways and allows for the identification of biomarkers for diseases. The unique feature of using GC-MS here lies in its ability to analyze complex biological samples effectively. While the advantages are clear, such as increased diagnostic power, there are challenges too, including sample complexity that can obscure data interpretation.
Proteomics
Proteomics, the large-scale study of proteins, also stands to benefit from advancements in GC-MS. Integrating this technique aids in characterizing proteins and their functions within biological systems. The significant characteristic of deploying GC-MS in proteomic studies is that it offers precise quantification and the ability to analyze structural variations of proteins.
One unique aspect of considering proteomics in tandem with GC-MS is the potential for multi-dimensional analysis. This helps researchers make correlated conclusions about protein expression and metabolic changes in diseases. However, the methodβs complexity may raise barriers to entry for those not well-versed in advanced mass spectrometric techniques.
Overall, the future perspectives of GC-MS are vast and varied. The ongoing technological advances and expanding applications reflect a discipline that is as dynamic as it is crucial. The balance of overcoming emerging challenges while embracing innovation is the key to harnessing the full potential of GC-MS in the years to come.
By understanding these future perspectives, stakeholders in research and industry can make informed decisions about how to best leverage GC-MS to address pressing scientific questions and societal needs.
Culmination
When it comes to understanding the multifaceted realm of gas chromatograph mass spectrometry (GC-MS), the conclusion serves as a pivotal element in wrapping up the extensive discussion on its significance in scientific exploration. GC-MS is not merely a technique; it's a powerful tool that crystallizes the fusion of gas chromatography and mass spectrometry, opening doors to precise analysis across numerous fields. It enables researchers to unravel complex molecules, making the invisible tangible.
Summary of Key Points
To encapsulate the insights gleaned from earlier sections, here are the key points regarding GC-MS:
- Integration of Techniques: GC-MS combines the separation capability of gas chromatography with the identification power of mass spectrometry. This synergy enhances analytical precision.
- Diverse Applications: Its relevance spans environmental analysis, clinical diagnostics, and pharmaceutical research. Each application showcases the strength of GC-MS in solving real-world problems.
- Challenges Faced: Despite its robustness, GC-MS faces hurdles like intricate sample preparation and calibration issues, which necessitate careful consideration for effective utilization.
- Future Directions: Advancements in technology promise exciting developments. Miniaturization and integration with other analytical techniques could revolutionize its application spectrum.
The Importance of GC-MS in Modern Science
The role of GC-MS in modern science cannot be overemphasized. This technology has become fundamental in various sectors:
- Enhanced Detection and Quantitation: With its high sensitivity and resolving power, GC-MS is often the method of choice for trace analysis of organic compounds, something particularly crucial in toxicology and forensic studies.
- Comprehensive Characterization: It allows scientists to identify, quantify, and characterize complex mixtures of compounds in a single analysis, facilitating more comprehensive insights.
- Driving Research Forward: As fields like metabolomics and proteomics burgeon, GC-MS stands at the forefront, enabling researchers to explore the intricate biochemical interactions that underpin life itself.
- Innovation and Development: Ongoing advancements in GC-MS technology pave the way for novel applications, making it an indispensable component of contemporary laboratories.
In reflection, GC-MS represents not only an analytical technique but a beacon of innovation that uplifts modern science. As research continues to evolve, so too will the potential of GC-MS to unravel the complexities of the natural world.
"GC-MS is an example of how combining techniques can yield greater understanding and breakthroughs in science rather than relying on singular methods."
Moving forward, the importance of thoroughly grasping both its capabilities and limitations is crucial for anyone engaged in research, analysis, or teaching. Armed with this knowledge, professionals can leverage GC-MS to its fullest, thus contributing to the advancement of science as a whole.